Vitamin D suppresses ferroptosis and protects against neonatal hypoxic-ischemic encephalopathy by activating the Nrf2/HO-1 pathway
Introduction
Neonatal hypoxic-ischemic encephalopathy (HIE) is a type of newborn brain damage caused by cerebral hypoxic ischemia, which is one of the main causes of neonatal death and disability worldwide (1). Surviving children with HIE often have neurological sequelae, such as epilepsy, mental retardation, and cerebral palsy, which can seriously affect their quality of life (2-4). While hypothermia is the only clinically available treatment method for HIE, only 50% of treatments are effective (3,5), and there is an urgent need to develop novel treatment approaches.
In recent years, vitamin D (VD) has received great attention as a neuroprotective drug in the treatment of neonatal HIE (6). VD is a pleiotropic steroid hormone that plays a crucial role in mineral balance, anti-inflammatory, immune response, and metabolic disease (7-9). Through producing antimicrobial peptides (e.g., cathelicidin), VD exerts a vital function in the innate immunity (7). Administration of VD can suppress inflammation response via decreasing the secretion of pro-inflammatory cytokines, such as interleukin (IL)-6 and tumor necrosis factor-α (TNF-α) (9). VD has also been demonstrated to have a protective role in the nervous system (6). VD deficiency is associated with poor brain development and nerve damage (10), and supplementation reduces cerebral infarction in animals (11). Moreover, most HIE infants have low serum VD concentration (<20 ng/mL) after birth, and VD insufficiency is associated with the severity of neonatal HIE (12). However, the exact function and molecular mechanism of VD in neonatal HIE is unclarified.
The development of HIE involves multiple pathological processes, including oxidative stress, excitotoxicity, inflammation, immune response, and cell death (13,14). While apoptosis and necrosis are two major causes of cell death in various types of brain injury, increasing studies suggest the involvement of ferroptosis in neurological disease, such as ischemic and hemorrhagic stroke, Alzheimer’s disease, and Parkinson’s disease (15,16). Ferroptosis is a newly discovered type of cell death, which differs from traditional programmed cell deaths, such as apoptosis and necrosis (17). Its main characteristic is iron accumulation and lipid peroxide generation, which ultimately lead to cell death (18). It has been reported that compared with the sham group, the reactive oxygen species (ROS) level and iron-associated proteins were increased and the expression of GSH and GPX4 was decreased in neonatal rats with hypoxic-ischemic brain damage (19), indicating that ferroptosis has a promoting effect on HIE. Accordingly, inhibiting ferroptosis can attenuate hippocampal HIE in neonatal rats (20). Together, these results suggest ferroptosis is an important mechanism accounting for HIE.
While a previous study reported VD as [1,25-(OH)2D3] had an inhibitory effect on ferroptosis in zebrafish liver cells (21), whether it prevents neonatal HIE through regulating ferroptosis is unknown. In the present study, we investigated the functional activity of VD in neonatal HIE and explored the underlying molecular mechanism. We present the following article in accordance with the ARRIVE reporting checklist (available at https://tp.amegroups.com/article/view/10.21037/tp-22-397/rc).
Methods
Hypoxic-ischemic brain damage (HIBD) model
An HIBD model was applied to investigate the effect of VD on HIE. Sprague-Dawley (SD) rats (male, 10–12 weeks old, 200–250 g) were bought from Southern Medical University and randomly divided into three groups: a control group, a HIBD group, and a HIBD + VD group (n=6). The HIBD model was constructed using the Rice-Vannucci method as reported previously (22). Briefly, rats were anesthetized, and their right common carotid artery was exposed and ligated. After recovery for 60 min at room temperature, rats were exposed to 8% oxygen balanced with 92% nitrogen at 37 ℃ for 2 h. Rats in the control group (n=6) underwent a neck incision with exploration of the right carotid artery, but without ligation or hypoxia. In the control and HIBD groups, rats received daily intraperitoneal injections of saline, while in the HIBD + VD group, they were intraperitoneally injected with VD (0.1 µg/kg) every day. After 2 weeks of treatment, rats were sacrificed, the brains removed, and cortical tissues were isolated and stored at −80 ℃ for further experiments. Animal experiments were performed under a project license (approval number: gdpulac 2021118) approved by the Institutional Animal Care and Use Committee of Guangzhou Medical University, in compliance with the guidelines of the Institutional Animal Care and Use Committee of Guangzhou Medical University. A protocol was prepared before the study without registration.
Hematoxylin-eosin (HE) staining and Nissl staining
For HE staining, paraffin sections of brain neuron tissues were stained with hematoxylin (Solarbio, Beijing, China) and eosin (Solarbio). Nissl staining was performed to monitor the change of motor neurons in brain tissues. Deparaffinized sections were hydrated with distilled water and stained with methyl violet solution for 15 min and differentiated with Nissl differentiation solution (Beyotime, Beijing, China) for 5 s. After dehydration, sections were mounted onto slides and the stained sections were observed under a light microscope (Zeiss, Jena, Germany).
Cell viability assay
Cell viability assay was used to determine the growth of SH-SY5Y cells in different groups [control, oxygen-glucose deprivation (OGD), OGD + VD]. Cells were purchased from ATCC (USA) and cultured in DMEM (Gibco, USA) containing 10% foetal bovine serum (FBS) and 1% penicillin-streptomycin (Gibco), and cell viability was assessed by Cell Counting Kit-8 (CCK-8) assay (Seyotin, Guangzhou, China) before seeding into 96-well plates. The OGD model was constructed as reported previously (23). In brief, after washing three times with phosphate buffer saline (PBS), cells were maintained in glucose-free DMEM under hypoxic conditions with 95% N2 and 5% CO2 at 37 ℃ for 6 h. Glucose was then changed to a normal level, and cells were incubated in an atmosphere with 5% CO2 at 37 ℃ for another 18 h. For VD treatment, 20 ng/mL VD was added to cells 24 h prior to OGD modeling, then CCK-8 solution was added and incubated for 1–4 h. Absorbance at 450 nm was detected by a microplate reader.
Quantitative real real-time PCR (qRT-PCR)
qRT-PCR was performed to determine mRNA expression. Total RNA was extracted from SH-SY5Y cells using TRIzol reagent (ThermoFisher Scientific, USA), then reverse-transcribed into cDNA using the PrimeScript RT reagent kit (Seyotin). qRT-PCR was performed using SYBR Green (Seyotin) according to the manufacturer’s introductions. The PCR conditions were as follows: 95 ℃, 2 min and 40 cycles of 30 s at 95 ℃, 30 s at 60 ℃, and 30 s at 68 ℃. The relative gene expression was calculated using the formula of 2−ΔΔCt and GAPDH was used as an internal control. The primer sequences were listed as follow: NRF2-F 5'-ATGCCTTCCTCTGCTGCCAT-3', NRF2-R 5'-CCGTGCCTTCAGTGTGCTTC-3'; HO-1-F 5'-GCCCACGCATATACCCGCTA-3', HO-1-R 5'-GTCGATGCTCGGGAAGGTGA-3'; GPX4-F 5'-ACGCCGAGTGTGGTTTACGA-3', GPX4-R 5'-TTTCCACAGTGGGTGGGCAT-3'.
Western blot analysis
Western blot was performed to examine the protein expression. Brain neuron tissues and SH-SY5Y cells were used for protein isolation, and after lysis in RIPA buffer, cell lysate was incubated at 4 ℃ for 20 min, followed by centrifugation for 20 min at 4 ℃. The supernatant was collected, and protein concentration was identified by BCA method (Seyotin). Protein was separated by sodium dodecyl sulfate polyacrylamide gel electrophoresis (SDS-PAGE) and transferred onto PDVF membranes which were blocked with 5% non-fat milk followed by incubation with primary antibody at 4 ℃ overnight. After washing, the membrane was incubated with second antibodies. The protein bands were detected by an ECL detection Kit (Seyotin), and the band intensity was quantified by ImageJ software. Antibodies used were anti-Nrf2 (Abcam, Cambridge, UK), anti-HO-1 (Abcam), anti-GPX4 (Abcam), anti-GAPDH (Cell Signaling Technology, Boston, USA), anti-TNF-α (Abcam) anti-IL-6 (Abcam), and anti-IL-1β (R&D Systems, Minneapolis, USA).
Immunofluorescence staining
Brain tissues and cells cultured on coverslip from different treatments were washed with PBS and fixed with 4% formaldehyde before incubation with 0.1% Triton X-100 for 20 min at room temperature. After three washes with PBS, samples were blocked with 5% bovine serum albumin (BSA), followed by incubation with primary antibody at 4 ℃ overnight. Samples were then incubated with fluorescence-labeled secondary antibodies (Alexa Fluor 488 goat anti-rabbit IgG or Alexa Fluor 594 goat anti-mouse IgG, 1:250, Thermo Fisher Scientific, USA), and 4',6-diamidino-2-phenylindole (DAPI) was utilized to stain the nucleus. Samples were observed under a fluorescence microscope.
Transmission electron microscopy
Tissue samples were washed with PBS three times and fixed with 2.5% glutaraldehyde for 2 h, then washed a further three times with PBS and fixed with 1% osmium acid for 2 h. After dehydration, samples were treated with a mixture of acetone and embedding agent (2:1) for 3 h, followed by a mixture of acetone and embedding agent (1:2) overnight. Samples were then treated with embedding agent for 2 h at 37 ℃. After being heated overnight at 70 ℃, samples were cut into 70 nm thick sections, placed on a copper mesh, and stained with 2% uranyl acetate and 4% lead citrate. The ultrastructure of samples was visualized under transmission electron microscopy.
ROS determination
The content of ROS in SH-SY5Y cells with different treatments was determined using an ROS fluorescent probe DCFH-DA (Solarbio). SH-SY5Y cells were collected and washed with PBS, and after fixing with 4% formaldehyde, were stained with DCFH-DA. After three washes with PBS, cells were stained with DAPI to visualize the nuclei, and were observed under a fluorescence microscope.
Superoxide dismutase (SOD), malondialdehyde (MDA), and glutathione (GSH) measurement
Brain tissues were collected from each group, and the MDA content and SOD activity were determined using the MDA Content Detection Kit and the SOD Activity Detection Kit (Beyotime Institute of Biotechnology, Nantong, Jiangsu, China) according to the manufacturer’s instructions, respectively. The GSH level was measured with a GSH detection assay kit (Nanjing Jiancheng Bioengineering Institute, Nanjing, China), following the manufacturer’s protocol.
Enzyme-linked immunosorbent assay (ELISA) assay
ELISA assay was conducted to detect the levels of TNF-α, IL-6, and IL-1β of each group following the manufacturer’s instructions (Bioswamp, Shanghai, China). All experiments were repeated three times.
Statistical analysis
All data are expressed as the mean ± standard deviation (SD). Comparations among more than two groups were performed using the one-way analysis of variance (ANOVA) with the Bonferroni post hoc test. A P value lower than 0.05 was considered statistically significant.
Results
VD attenuates neonatal HIE in rats
Gross brain pathology analysis revealed treatment with VD attenuated brain edema (Figure 1A), and Nissl staining showed the number of neurons in brain tissues of the HIBD + VD group was greater than the HIBD group (Figure 1B). HE staining showed clear and normal cell contour in brain neuron tissue in the control group, whereas in the HIBD group, tissue was atrophied, the cortex was thin, and gaps were formed. However, in the HIBD + VD group, the damage of brain tissues was ameliorated compared with the HIBD group (Figure 1C). Together, these results indicate VD ameliorates brain histologic damage.
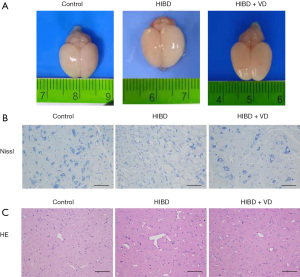
VD suppresses HI-induced mitochondria and oxidative damage
Mitochondria and oxidative damage are important mechanisms accounting for brain injury. As shown in Figure 2A, while mitochondria injury was increased in the HIBD group, treatment with VD reduced this damage. To assess the effect of VD on HI-induced oxidative damage, MDA content, SOD activity, and GSH level were measured, and the results showed the content of MDA was significantly induced in HI-treated rats, but treatment with VD markedly decreased this induction (Figure 2B). Compared with the control group, the SOD activity and GSH level were obviously reduced in the HIBD group but were significantly increased in the HIBD + VD group (Figure 2C,2D). Together, these results suggest VD has an inhibitory effect on HI-induced mitochondria and oxidative damage.
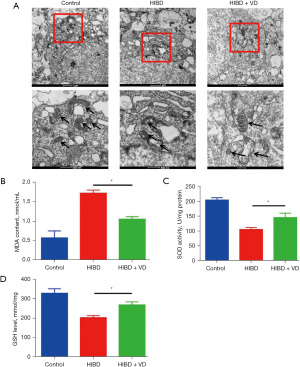
VD inhibits HI-induced ferroptosis by increasing NRF2/HO-1 expression
Nrf2 and HO-1 are important regulators implicated in ferroptosis, and we investigated whether VD could affect their expressions. The mRNA levels of Nrf2 and HO-1 were significantly increased in the HIBD + VD group compared with the HIBD group, and the mRNA level of GPX4 was higher in the HIBD + VD group than in the HIBD group (Figure 3A-3C). Consistently, immunofluorescence staining and Western blot showed the protein expressions of Nrf2 and GPX4 were markedly increased in HI-treated rats following VD treatment (Figure 3D-3G). Collectively, these results indicate VD-induced Nrf2/HO-1 expression contributes to ferroptosis suppression.
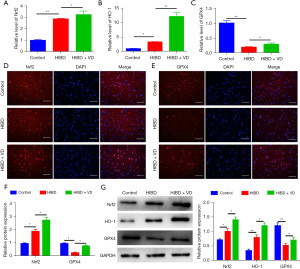
VD enhances cell viability and inhibits oxidative response in OGD-treated cells
To further confirm the effect of VD on oxidation response, an OGD model on SH-SY5Y cells was established. CCK-8 assay showed the viability of SH-SY5Y cells were significantly inhibited after OGD treatment, whereas administration of VD restored cell capability (Figure 4A). In OGD-treated SH-SY5Y cells, the content of MDA was highly increased, and VD treatment reversed this effect (Figure 4B), while in comparison with control cells, GSH level and SOD activity were markedly reduced in oxygen glucose deprivation-treated cells (Figure 4C,4D). However, exposure to VD elevated GSH level and SOD activity in OGD-treated cells (Figure 4C,4D). Immunofluorescence staining showed the intensity of ROS was elevated in OGD-treated SH-SY5Y cells, while administration of VD counteracts this enhancement (Figure 4E). Collectively, these results indicate VD treatment has a suppressive effect on OGD-induced oxidation stress.
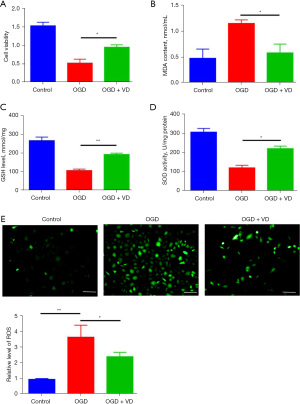
VD represses OGD-induced ferroptosis by upregulation of Nrf2/HO-1
An OGD model was used to verify the effect of VD on Nrf2/HO-1 expression. qRT-PCR analysis showed the mRNA levels of Nrf2, HO-1, and GPX4 were increased in the OGD + VD group compared with the OGD group (Figure 5A-5C), and Western blot also showed that the protein levels of Nrf2 and HO-1 were elevated in the OGD + VD group compared with the OGD group (Figure 5D). Immunofluorescence staining also showed the content of GPX4 was higher in the OGD + VD group than in the OGD group (Figure 5E). Together, these data indicate VD exerts a suppressive effect on OGD-induced ferroptosis by activating the Nrf2/HO-1 pathway.
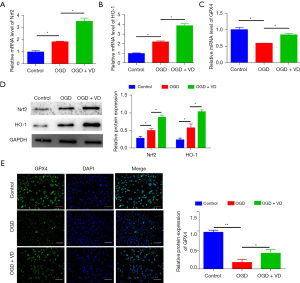
VD reduces OGD-induced inflammation by upregulation of NRF2
In agreement with the results in vivo, ELISA assay and Western blot analysis showed the levels of TNF-α, IL-6, and IL-1β were significantly increased in the OGD group, compared with the control group (Figure 6). However, VD treatment markedly reduced HI-mediated elevation of TNF-α, IL-6, and IL-1β in SH-ST5Y cells. Together, these data indicate upregulation of Nrf2 contributes to VD-mediated suppression of OGD-induced inflammation.
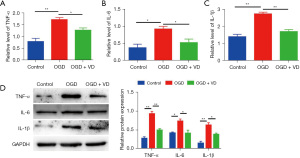
Discussion
Current therapeutic treatments for HIE are unsatisfactory, and great efforts are required to improve the treatment efficiency of this major threat to neonatal life. In this study, we demonstrated VD had a protective role in neonatal HIE, and our data revealed the underlying mechanism of VD in HIE is mediated by inhibition of ferroptosis via activation of the Nrf2/HO-1 pathway.
VD is associated with many functions in the body, and is a potent neurohormone with an important role in neurologic disease (24). VD deficiency is considered a risk factor for abnormal brain development (25,26), and neonates are at high risk, which may be due to the high prevalence of maternal deficiency during pregnancy (6). Multiple preclinical studies have revealed infants born to VD-deficient mothers are at a high risk of developing neonatal brain injury, and recent clinical studies have shown that VD deficiency in neonates is associated with HIE (6,10,27,28). Lowe et al. reported VD exposure improved outcomes in neonatal hypoxic ischemic rats treated with N-acetylcysteine and hypothermia (22), and consistently, in the present study, our data revealed VD treatment ameliorated neonatal HIE in rats and in OGD-treated cells. When examining the status of VD and oxidation in neonates with neonatal HIE, the results show that VD levels were statistically lower and MDA levels were significantly higher in infants with HIE (29). In agreement with this result, we found VD treatment highly decreased HI-induced MDA content and increased GSH level and SOD activity which were inhibited by HIE. The production of ROS has been involved in many mechanisms of cellular injury (6), and it has been reported that calcitriol [1α,25-(OH)2D3], an active form of VD, inhibits the formation of ROS by affecting the hydrogen peroxide pathway (30). Herein, we also found VD treatment reduced ROS induced by HI.
Previous studies have demonstrated VD can change free radical formation via the metabolism of metal ions, including iron. Cellular accumulation of ferric iron results in increased ROS formation via the Fenton reaction (31). It has been reported that calcitriol prevents neuronal cell injury by inhibiting iron-associated oxidation (32). Ferroptosis is an iron-dependent cell death thought to play a major role in neurodegenerative diseases, including HIE (16,18-20), and targeting ferroptosis is considered a promising approach for treatment (33). In this study, we found VD to be a negative regulator of ferroptosis.
Ferroptosis is primary triggered by a failure of the GSH-GPX4 reductive system and associated overwhelming lipid peroxidation (34). Consistently, our data showed OGD-treatment decreased GSH, SOD, and GPX4 levels, indicating an induction of ferroptosis in the HIE model. In contrast, treatment with VD reversed the inhibitory effect of HI on GSH, SOD, and GPX4 contents. To explore the underlying mechanism of VD in ferroptosis, we found VD could increase the expression of Nrf2 and HO-1. Previous studies have demonstrated Nrf2/HO-1 are critical factors regulating the antioxidant response (35), as well as key regulators of the neuroprotection response (36). Gao et al. reported that upregulation of Nrf2 and HO-1 expression relieves oxidative stress and alleviates HIE in neonatal rats (37). Results from Deng group also showed that lycium ruthenicum polysaccharide 3 (LRP3) protects cortical neurons against neonatal HIE via activation of Nrf2/HO-1 pathway (38). In addition, Nrf2/HO-1 pathway plays an important role in ferroptosis. It has been reported that etomidate inhibits myocardial ischemia/reperfusion-induced ferroptosis via the Nrf2/HO-1 pathway (39). Gastrodin prevents glutamate-induced ferroptosis through Nrf2/HO-1 signaling (40), and in this study, we found VD increased Nrf2/HO-1 expression, which resulted in suppressed ferroptosis in neonatal HIE rats.
In conclusion, using both in vivo and in vitro models, we found VD could efficiently alleviate neonatal HIE in rats. Mechanistically, VD repressed ferroptosis and ameliorated mitochondria and oxidative damage via the Nrf2/HO-1 pathway. Our study provides insights into a novel mechanism by which VD protects against neonatal HIE, implying it may serve as a promising agent for HIE therapy.
Acknowledgments
Funding: This study was supported by funding from the Guangzhou Women and Children’s Medical Center (No. GWCMC2020-4-016).
Footnote
Reporting Checklist: The authors have completed the ARRIVE reporting checklist. Available at https://tp.amegroups.com/article/view/10.21037/tp-22-397/rc
Data Sharing Statement: Available at https://tp.amegroups.com/article/view/10.21037/tp-22-397/dss
Conflicts of Interest: All authors have completed the ICMJE uniform disclosure form (available at https://tp.amegroups.com/article/view/10.21037/tp-22-397/coif). The authors have no conflicts of interest to declare.
Ethical Statement: The authors are accountable for all aspects of the work in ensuring that questions related to the accuracy or integrity of any part of the work are appropriately investigated and resolved. Animal experiments were performed under a project license (approval number: gdpulac 2021118) approved by the Institutional Animal Care and Use Committee of Guangzhou Medical University, in compliance with the guidelines of the Institutional Animal Care and Use Committee of Guangzhou Medical University.
Open Access Statement: This is an Open Access article distributed in accordance with the Creative Commons Attribution-NonCommercial-NoDerivs 4.0 International License (CC BY-NC-ND 4.0), which permits the non-commercial replication and distribution of the article with the strict proviso that no changes or edits are made and the original work is properly cited (including links to both the formal publication through the relevant DOI and the license). See: https://creativecommons.org/licenses/by-nc-nd/4.0/.
References
- Rodríguez M, Valez V, Cimarra C, et al. Hypoxic-Ischemic Encephalopathy and Mitochondrial Dysfunction: Facts, Unknowns, and Challenges. Antioxid Redox Signal 2020;33:247-62. [Crossref] [PubMed]
- Badawi N, Felix JF, Kurinczuk JJ, et al. Cerebral palsy following term newborn encephalopathy: a population-based study. Dev Med Child Neurol 2005;47:293-8. [Crossref] [PubMed]
- Lugli L, Balestri E, Berardi A, et al. Brain cooling reduces the risk of postneonatal epilepsy in newborns affected by moderate to severe hypoxic-ischemic encephalopathy. Minerva Pediatr (Torino) 2021;73:150-8. [Crossref] [PubMed]
- WHITE HH. FOWLER FD. Chronic lead encephalopathy. A diagnostic consideration in mental retardation. Pediatrics 1960;25:309-15. [PubMed]
- Lemyre B, Chau V. Hypothermia for newborns with hypoxic-ischemic encephalopathy. Paediatr Child Health 2018;23:285-91. [Crossref] [PubMed]
- Stessman LE, Peeples ES. Vitamin D and Its Role in Neonatal Hypoxic-Ischemic Brain Injury. Neonatology 2018;113:305-12. [Crossref] [PubMed]
- Bartley J. Vitamin D: emerging roles in infection and immunity. Expert Rev Anti Infect Ther 2010;8:1359-69. [Crossref] [PubMed]
- Park CY, Han SN. The Role of Vitamin D in Adipose Tissue Biology: Adipocyte Differentiation, Energy Metabolism, and Inflammation. J Lipid Atheroscler 2021;10:130-44. [Crossref] [PubMed]
- Sudjaritruk T, Bunupuradah T, Aurpibul L, et al. Impact of Vitamin D and Calcium Supplementation on Bone Mineral Density and Bone Metabolism Among Thai Adolescents With Perinatally Acquired Human Immunodeficiency Virus (HIV) Infection: A Randomized Clinical Trial. Clin Infect Dis 2021;73:1555-64. [Crossref] [PubMed]
- Hagag AA, El Frargy MS, Abd El-Latif AE. Vitamin D as an Adjuvant Therapy in Neonatal Hypoxia: Is it Beneficial? Endocr Metab Immune Disord Drug Targets 2019;19:341-8. [Crossref] [PubMed]
- Bao GQ, Yu JY. Vitamin D3 promotes cerebral angiogenesis after cerebral infarction in rats by activating Shh signaling pathway. Eur Rev Med Pharmacol Sci 2018;22:7069-77. [PubMed]
- Lowe DW, Hollis BW, Wagner CL, et al. Vitamin D insufficiency in neonatal hypoxic-ischemic encephalopathy. Pediatr Res 2017;82:55-62. [Crossref] [PubMed]
- Liu F, McCullough LD. Inflammatory responses in hypoxic ischemic encephalopathy. Acta Pharmacol Sin 2013;34:1121-30. [Crossref] [PubMed]
- Allen KA, Brandon DH. Hypoxic Ischemic Encephalopathy: Pathophysiology and Experimental Treatments. Newborn Infant Nurs Rev 2011;11:125-33. [Crossref] [PubMed]
- Wu Y, Song J, Wang Y, et al. The Potential Role of Ferroptosis in Neonatal Brain Injury. Front Neurosci 2019;13:115. [Crossref] [PubMed]
- Weiland A, Wang Y, Wu W, et al. Ferroptosis and Its Role in Diverse Brain Diseases. Mol Neurobiol 2019;56:4880-93. [Crossref] [PubMed]
- Yu H, Guo P, Xie X, et al. Ferroptosis, a new form of cell death, and its relationships with tumourous diseases. J Cell Mol Med 2017;21:648-57. [Crossref] [PubMed]
- Li S, Huang Y. Ferroptosis: an iron-dependent cell death form linking metabolism, diseases, immune cell and targeted therapy. Clin Transl Oncol 2022;24:1-12. [Crossref] [PubMed]
- Lin W, Zhang T, Zheng J, et al. Ferroptosis is Involved in Hypoxic-ischemic Brain Damage in Neonatal Rats. Neuroscience 2022;487:131-42. [Crossref] [PubMed]
- Zhu K, Zhu X, Sun S, et al. Inhibition of TLR4 prevents hippocampal hypoxic-ischemic injury by regulating ferroptosis in neonatal rats. Exp Neurol 2021;345:113828. [Crossref] [PubMed]
- Cheng K, Huang Y, Wang C. 1,25(OH)2D3 Inhibited Ferroptosis in Zebrafish Liver Cells (ZFL) by Regulating Keap1-Nrf2-GPx4 and NF-κB-hepcidin Axis. Int J Mol Sci 2021;22:11334. [Crossref] [PubMed]
- Lowe DW, Fraser JL, Rollins LG, et al. Vitamin D improves functional outcomes in neonatal hypoxic ischemic male rats treated with N-acetylcysteine and hypothermia. Neuropharmacology 2017;123:186-200. [Crossref] [PubMed]
- Fan Z, Cai L, Wang S, et al. Baicalin Prevents Myocardial Ischemia/Reperfusion Injury Through Inhibiting ACSL4 Mediated Ferroptosis. Front Pharmacol 2021;12:628988. [Crossref] [PubMed]
- Kalueff AV, Eremin KO, Tuohimaa P. Mechanisms of neuroprotective action of vitamin D(3). Biochemistry (Mosc) 2004;69:738-41. [Crossref] [PubMed]
- Eyles D, Brown J, Mackay-Sim A, et al. Vitamin D3 and brain development. Neuroscience 2003;118:641-53. [Crossref] [PubMed]
- McGrath JJ, Féron FP, Burne TH, et al. Vitamin D3-implications for brain development. J Steroid Biochem Mol Biol 2004;89-90:557-60. [Crossref] [PubMed]
- Kumar J, Muntner P, Kaskel FJ, et al. Prevalence and associations of 25-hydroxyvitamin D deficiency in US children: NHANES 2001-2004. Pediatrics 2009;124:e362-70. [Crossref] [PubMed]
- Wagner CL, Greer FRAmerican Academy of Pediatrics Section on Breastfeeding, et al. Prevention of rickets and vitamin D deficiency in infants, children, and adolescents. Pediatrics 2008;122:1142-52. [Crossref] [PubMed]
- Mutlu M, Sarıaydın M, Aslan Y, et al. Status of vitamin D, antioxidant enzymes, and antioxidant substances in neonates with neonatal hypoxic-ischemic encephalopathy. J Matern Fetal Neonatal Med 2016;29:2259-63. [PubMed]
- Ibi M, Sawada H, Nakanishi M, et al. Protective effects of 1 alpha,25-(OH)(2)D(3) against the neurotoxicity of glutamate and reactive oxygen species in mesencephalic culture. Neuropharmacology 2001;40:761-71. [Crossref] [PubMed]
- Mello-Filho AC, Meneghini R. Iron is the intracellular metal involved in the production of DNA damage by oxygen radicals. Mutat Res 1991;251:109-13. [Crossref] [PubMed]
- Baudet C, Chevalier G, Chassevent A, et al. 1,25-Dihydroxyvitamin D3 induces programmed cell death in a rat glioma cell line. J Neurosci Res 1996;46:540-50. [Crossref] [PubMed]
- Vitalakumar D, Sharma A, Flora SJS. Ferroptosis: A potential therapeutic target for neurodegenerative diseases. J Biochem Mol Toxicol 2021;35:e22830. [Crossref] [PubMed]
- Shui S, Zhao Z, Wang H, et al. Non-enzymatic lipid peroxidation initiated by photodynamic therapy drives a distinct ferroptosis-like cell death pathway. Redox Biol 2021;45:102056. [Crossref] [PubMed]
- Diao C, Chen Z, Qiu T, et al. Inhibition of PRMT5 Attenuates Oxidative Stress-Induced Pyroptosis via Activation of the Nrf2/HO-1 Signal Pathway in a Mouse Model of Renal Ischemia-Reperfusion Injury. Oxid Med Cell Longev 2019;2019:2345658. [Crossref] [PubMed]
- Minj E, Yadav RK, Mehan S. Targeting Abnormal Nrf2/HO-1 Signaling in Amyotrophic Lateral Sclerosis: Current Insights on Drug Targets and Influences on Neurological Disorders. Curr Mol Med 2021;21:630-44. [Crossref] [PubMed]
- Gao Y, Fu R, Wang J, et al. Resveratrol mitigates the oxidative stress mediated by hypoxic-ischemic brain injury in neonatal rats via Nrf2/HO-1 pathway. Pharm Biol 2018;56:440-9. [Crossref] [PubMed]
- Deng K, Li Y, Xiao M, et al. Lycium ruthenicum Murr polysaccharide protects cortical neurons against oxygen-glucose deprivation/reperfusion in neonatal hypoxic-ischemic encephalopathy. Int J Biol Macromol 2020;158:562-8. [Crossref] [PubMed]
- Lv Z, Wang F, Zhang X, et al. Etomidate Attenuates the Ferroptosis in Myocardial Ischemia/Reperfusion Rat Model via Nrf2/HO-1 Pathway. Shock 2021;56:440-9. [PubMed]
- Jiang T, Cheng H, Su J, et al. Gastrodin protects against glutamate-induced ferroptosis in HT-22 cells through Nrf2/HO-1 signaling pathway. Toxicol In Vitro 2020;62:104715. [Crossref] [PubMed]
(English Language Editor: B. Draper)