Preoperative evaluation and surgical decision-making in pediatric epilepsy surgery
Introduction
Epilepsy is a common yet rarely discussed chronic disease. In the United States, 5.1 million individuals carry the diagnosis of a seizure disorder or epilepsy (1-3). In 2012, direct medical cost of epilepsy treatment amounted to $9.6 billion (4); the cost of community services, lost wages of individuals and caregivers, and immense social stress of patients and their families add to the toll. The age of onset of epilepsy is bimodal. Of the 5.1 million Americans with a seizure disorder or epilepsy, 460,000 are age 17 or younger and are actively undergoing epilepsy treatment (2,3). Underlying etiologies of epilepsy include neonatal hypoxia, congenital anomalies, traumatic brain injury, meningitis, brain tumor, and stroke. Frequently, however, an underlying cause is never identified.
Several modalities for epilepsy treatment exist. Medical management in the form of antiepileptic drugs (AEDs) is the first line of treatment and is successful in seizure control in 60–80% of cases (5). At present, the United States Food and Drug Administration (FDA) lists 18 AEDs, with several drugs having short-acting and extended-release preparations. Drug-resistant epilepsy (DRE) is defined by the International League Against Epilepsy (ILAE) as an adequate trial of two or more appropriately selected AEDs, alone or in combination, with failure of treatment resulting in continued seizures or intolerable side effects (6).
Uncontrolled seizures represent an important public health problem. A recent study published by Berg et al. (7) prospectively analyzed 198 children based on age of first seizure, pharmacoresistance, and cognitive outcomes. Patients with DRE had statistically worse processing speed and verbal comprehension scores, freedom from distractibility, and overall intelligence quotients (IQs). Furthermore, among children with DRE, younger age of seizure onset correlated negatively with IQ. As these children age, continued epileptic activity can prevent reaching major social milestones, including driving and living independently.
Options for the treatment of DRE include lifestyle modification and surgery. The ketogenic diet, consisting of a combination of high fat and low carbohydrate content, has been used to treat DRE with some success. It is the first-line treatment for patients with glucose transporter type 1 and pyruvate dehydrogenate complex deficiencies (8,9). The ketogenic diet’s success depends on the ability of the patient to maintain it. Among 59 patients initially enrolled in a recent study, only 24 were able to maintain the ketogenic diet for 12 months. Although only a minority of patients were able to sustain the diet for the entire study, 21 of those 24 children experienced at least a 50% reduction in seizure frequency.
Independent of the ketogenic diet, children with persistent seizures (due to failure to control seizures or intolerable medication side effects) should be referred for surgical evaluation as soon as they meet ILAE criteria for DRE (10). Timing is critical because of the impact of persistent seizures on the developing brain and because of the greater neuroplasticity in younger children. If surgical intervention necessitates resection or disconnection of eloquent areas of brain and loss of function, potential for reorganization is optimal at younger ages. Furthermore, continued epileptic activity can lead to the spread of seizure activity to previously normal areas of brain, an effect called “kindling”, leading to further neurologic deficit and multifocal epilepsy, which may be less amenable to surgical cure. Early patient assessment is critical, especially in patients with developmental delay. The factor most consistently associated with seizure freedom is the ability to completely remove the epileptogenic zone (11-13), which can often be identified through precise preoperative planning.
Preoperative evaluation
There are several components to a thorough presurgical evaluation. The core components include a detailed medical history, physical examination, electroencephalography (EEG), and structural brain magnetic resonance imaging (MRI). Other noninvasive tests including magnetoencephalography (MEG) and metabolic studies are often complementary. In the context of discordant data from noninvasive studies, epileptic foci near eloquent cortex, or in patients with a normal structural MRI, intracranial EEG recordings with subdural electrode arrays and depth electrodes may also be required.
Electroencephalography
Ictal scalp EEG can be useful for localization of an epileptic zone as it is widely available and relatively cost effective. Among children with DRE and normal structural findings MRI, EEG is critical for localization of the epileptic zone or at least hemispheric lateralization. Scalp EEG is less sensitive for epileptic foci located in the interhemispheric, basal, or mesial temporal locations. In addition, although interictal signal abnormalities may exist either remote from a cortical lesion or in a multifocal pattern, this should not influence eligibility or extent of resection if a solitary structural lesion is demonstrated by MRI (14-16). Video EEG is also useful in capturing and verifying auras. For example, forced turning of the head and eyes with neck extension localizes to the contralateral frontal eye fields and can be the first indication of frontal lobe epilepsy (17). Video EEG is also helpful in confirming whether stereotypic, episodic behaviors are a manifestation of seizure activity or are nonepileptic. If rapid seizure spread is present, localization of the initial epileptic zone may be limited, and additional modalities may be necessary to augment localization. In specific regard to infantile spasms, video EEG should be of sufficient length to capture wakefulness, sleep, and wakening (18); 24-hour video EEG monitoring has the best chance of capturing epileptic spasms and detecting hypsarrhythmia (19).
Magnetic resonance imaging
The Pediatric Epilepsy Surgery Task Force created by the ILAE recently published guidelines for evaluation of surgical candidates. Of all the proposed testing, only two modalities were uniformly agreed-upon core tests: scalp EEG and MRI. A single lesion on MRI corresponding to an EEG epileptogenic focus is a common situation with a potentially straightforward surgical plan, but more complex scenarios are frequently encountered. For example, in patients with tuberous sclerosis complex, several cortical tubers may be present on imaging, making it more difficult to determine the epileptogenic lesion noninvasively. In addition, adult patterns of myelination do not appear until 18 months of age (20), and it is often difficult to resolve subtle abnormalities including focal cortical dysplasia in infants. Finally, while MRI is a widely available diagnostic modality, barriers do exist. Strict contraindications include cochlear implants and cardiac pacemakers, and images can be degraded by presence of dental braces, ventricular shunts, and any patient motion. Any image degradation can be detrimental when attempting to detect subtle abnormalities on high-resolution scans.
Functional MRI (fMRI) is another tool frequently employed in the evaluation of patients with epilepsy. Classically, fMRI has been used to map motor, speech, auditory, and visual areas with the goal of avoiding them during surgical resection. Areas of activation are identified by blood-oxygen-level-dependent (BOLD) response or increased blood flow to an identified region while performing a specific task. It is critical that the specific tasks used during fMRI are appropriate to the age and education level of the patient, as responses are partially dependent on this (21) and responses may be attenuated by tasks that are too simple or too complex (22,23). Functional MRI is limited in terms of seizure localization, as only a few case reports exist of patients incidentally having a seizure while undergoing BOLD sequence imaging. More commonly, reflexive seizures, those that occur following a stimulus, such as flashing lights or excessive heat, can be provoked during imaging for localization purposes, as increased blood flow will be present to the epileptogenic region. The simultaneous use of scalp EEG and fMRI to localize interictal discharges was successful in identifying the epileptogenic region in 60% of cases in one report (24).
Magnetoencephalography
MEG is a more recent noninvasive method of epileptic focus identification. This imaging modality detects magnetic fields produced by the brain’s electrical activity. The signal is created by cortical dendrites and is best detected when oriented tangentially to the skull’s surface; signals from deeper structures are not detected as well as those from more superficial cortical regions. This modality is limited by regional availability and cost, but has some advantages in comparison to EEG. First, MEG is more sensitive than EEG in detecting smaller epileptic foci, with a threshold of 4–8 vs. 10–15 cm2 for scalp EEG (25). Interictal MEG epileptiform discharges, including activity from insular cortex (26-28), may be seen in approximately 50% of patients without detectable interictal epileptiform discharges on scalp EEG (29-31), which makes MEG particularly useful in guiding the placement of intracranial electrodes in patients without a structural lesion detectable by MRI. It is also advantageous compared with scalp EEG recordings for the detection and lateralization of interhemispheric epileptiform activity (32-34) and can detect interictal mesial temporal lobe discharges in as many as 85% of patients with mesial temporal lobe epilepsy (35). In summary, MEG may provide critical noninvasive localizing data when this cannot be done with EEG and MRI alone (36).
Positron emission tomography (PET)
PET using fluorine 18 fluorodeoxyglucose (FDG) is a study of brain metabolism and has a role in epileptogenic focus localization, especially when structural MRI is unrevealing. The brain’s primary energy source is glucose, and the labeled glucose may reveal areas of relative hypo- or hyper-metabolism with PET imaging. Hypometabolism occurs at an epileptic focus in an interictal state and is the result of neuronal loss, decreased synaptic activity, or decreased activity of blood–brain barrier glucose transport receptors (37-39). Because of the typical infrequency of seizure activity, the test is typically performed in an interictal state, but EEG is usually performed concurrently to determine whether seizure activity occurs during the examination. If a seizure occurs during the study, the EEG can correlate areas of possible glucose hypermetabolism and the epileptic focus. One limitation of FDG-PET imaging is that the area of glucose hypometabolism, or functional deficit zone, is often larger than the focal epileptic zone (40). PET localization plays the greatest role in MRI-occult epilepsy or in children with discordant noninvasive data between MRI and EEG studies. It is critical to note that regions of hypometabolism cannot differentiate the primary epileptogenic zone from secondary foci (41).
Single-photon emission computed tomography (SPECT)
SPECT is a noninvasive, metabolic imaging study similar to PET, with the distinction that it can be performed peri-ictally. Ictal SPECT is completed by injection of a radioisotope at the time of seizure onset. To conduct the study, children are admitted to the hospital, and long-term video EEG is placed. A seizure is confirmed by EEG and at that time the radioisotope is injected, and imaging is completed within subsequent hours. Interictal imaging is completed at a separate time for digital subtraction to identify areas of hypermetabolism during seizure onset. If SPECT and PET localization are concordant with EEG and MRI results, seizure freedom after surgery is more likely than if these studies are discordant (42). However, technical details may confound interpretation of the results, including the length of time between seizure onset and radioisotope administration, during which propagation of the seizure may occur. Children with focal cortical dysplasia, including those with a “normal” structural MRI, have higher rates of seizure freedom with complete resection of the perfusion abnormality identified by SPECT (43).
Intracranial electroencephalography (EEG)
Intracranial EEG is an invasive measure to identify an epileptic zone that incorporates subdural EEG strip, grid, and depth electrode placement via craniotomy and stereotactic depth electrode insertion (Figure 1). Intracranial EEG is often required if lateralization or localization of an epileptic focus has not been identified using noninvasive methods and also facilitates cortical stimulation mapping of functionally eloquent cortex. Although this modality is the gold standard of epileptic focus localization, limitations exist. For example, general anesthesia, the definitive treatment of status epilepticus, is required for intracranial electrode placement and has a variable impact on seizure activity (44).
Stereo EEG (SEEG), which involves the placement of multiple (often bilateral) depth electrodes, is commonly used in children in whom accurate lateralization or localization cannot be achieved with noninvasive diagnostic means. In one report from a single institution, 18 children underwent depth electrode placement because scalp EEG results were unclear or indicated discordant localization of an epileptic focus. An epileptic zone was identified in 15/18 patients, and resection of an area encompassing the epileptic zone plus 5 mm in all directions was undertaken without further electrocorticography. All patients who underwent resection were seizure-free at the one-year time point (45). Subdural strip electrodes have a similar goal of lateralization or localization but can be placed through burr holes only. Regardless of the technique, intracranial surveys often require subsequent craniotomy and grid placement for more precise localization once regional seizure onset is identified.
Craniotomy with insertion of large subdural electrode arrays requires general localization of the epileptic zone, either based on concordant noninvasive data or from a previous intracranial survey operation. Various electrode combinations are available, including dense grid electrodes (5 mm between platinum contacts) or double-sided interhemispheric grid electrodes. In certain cases, interictal recordings under total intravenous anesthesia in the operating room are sufficient to make surgical decisions. Frequently, however, staged craniotomy with long-term seizure monitoring to capture ictal onset and allow cortical stimulation mapping of functional eloquent cortex is required (46,47). Nearly two decades ago, Davies and colleagues described their experience using MRI to evaluate subdural and depth electrodes without complication (48). At our institution, an MRI is completed on postoperative day 1 to verify grid location and to identify possible surgical complications including hemorrhage or ischemia (Figure 1).
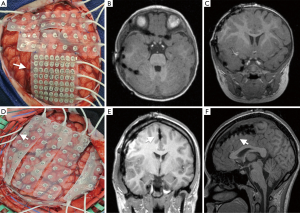
The advantages to grid placement include dense electrode coverage in the absence of a structural lesion identified by MRI, resolution of discordant noninvasive testing, evaluating the relationship of a structural lesion to an epileptic zone, evaluation of patients with dual pathology or multifocal epilepsy, and extraoperative awake cortical stimulation mapping to identify primary cortex and map eloquent function (49). The risks of staged craniotomy and placing large electrode arrays include intracranial hemorrhage, compression of cortical vascular structures causing cerebral edema and ischemia, as well as cerebrospinal fluid leak and meningitis. Large single-center series report complication rates between 10% and 20% (50,51).
Surgical decision-making
Epilepsy surgery requires a multidisciplinary team including a pediatric epileptologist, a pediatric neurosurgeon, and a neuropsychologist. Pediatric neuroradiologists, critical care specialists, behavioral health specialists, and a program coordinator are extremely valuable adjuncts. Dedicated pediatric epilepsy surgery conferences for detailed review of all component tests of the presurgical evaluation together with multidisciplinary clinics facilitate optimizing surgical decisions and communication with patients and families. Prior to surgical intervention, neuropsychological evaluation is mandatory. Neuropsychologists provide objective, baseline testing to identify individual cognitive strengths and deficits, anticipate postoperative deficits, and provide information regarding postoperative rehabilitation and education (25).
Because the primary goal of epilepsy surgery in children is seizure freedom, the previous section concentrated on methods for localizing an epileptic focus in patients with partial seizures with the goal of resection of the epileptogenic zone. Secondary goals of epilepsy surgery include palliation by decreasing seizure frequency and improving quality of life, cognitive development, and functional independence.
Focal lesions
In the context of a discrete lesion on MRI with a corresponding epileptic focus on EEG, the goal is typically gross total resection of the lesion. If suspicion exists that the ictal focus may extend beyond the borders of the MRI abnormality, further testing with intracranial EEG is possible. This may also be desirable if the lesion is near eloquent cortex. Noninvasive functional mapping such as fMRI is often useful to localize eloquent function preoperatively and determine whether cortical stimulation mapping is required. In younger children, task-based fMRI may be especially challenging. A wide range of heterogeneous pathology may represent the ictal substrate in the setting of a solitary structural lesion such as focal cortical dysplasia, brain tumors including World Health Organization grade 1 developmental tumors such as ganglioglioma or dysembryoplastic neuroepithelial tumors (DNET), vascular lesions including cavernous malformations and arteriovenous malformations, and postinfectious etiologies and perinatal insults (25,52-55).
Hypothalamic hamartomas, which are usually characterized clinically as gelastic seizures, are other discrete lesions encountered in children with DRE. EEG may demonstrate different patterns of focal or generalized seizure onset, but disconnection or resection of the hamartoma usually confers seizure freedom regardless of the patterns on the EEG. Previous microsurgical and endoscopic treatments for hypothalamic hamartoma have carried high morbidity, but over the past several years, the combination of laser ablation and disconnection with real-time MR thermography has emerged as a minimally invasive treatment option associated with excellent preliminary results (56).
Lobar lesions
Mesial temporal sclerosis is a frequent cause of DRE in adults, but less commonly identified in children. When it occurs in the pediatric population, it is most frequently in older children and adolescents (57-59). In younger children, temporal lesions including cortical dysplasia and developmental tumors like ganglioma and DNET are more common. When younger children present with mesial temporal sclerosis, dual pathology should be suspected and carefully investigated (25). In patients with a larger, more diffuse epileptogenic zone, including poorly defined cortical dysplasia as well as DRE with a normal MRI, larger regions of resection may be considered, including a lobar resection extending to the borders of primary cortex. This poses minimal risk beyond simple lesion removal if the anterior temporal and frontal lobes are involved, especially in the nondominant hemisphere. Careful functional and seizure mapping is mandatory when eloquent areas, including primary vision, language, or motor cortex, are adjacent to the ictal onset zone or possibly involved (25).
Hemispheric and multifocal lesions
Disease affecting an entire cerebral hemisphere requires special consideration. Many patients present with catastrophic epilepsy, developmental delay, and focal neurologic deficits including hemiparesis and hemianopsia. Disconnection of the dysfunctional hemisphere will prevent further seizure propagation and allow for stable and possibly improved function of the contralateral hemisphere. Hemispheric dysplasia such as hemimegalencephaly, encephalomalacia in the context of remote middle cerebral artery infarction, Sturge-Weber syndrome, and Rasmussen encephalitis are common causes of DRE that may require hemispherectomy (60,61). Patients with pre-existing focal manifestations of hemispheric dysfunction, including hemiplegia and hemianopia, may not need additional testing if scalp EEG and MRI are convincing of a dysfunctional hemisphere, especially if it is the nondominant hemisphere. Extensive presurgical evaluation should be conducted in patients with less severe neurologic deficits, and preoperative functional mapping of language function is mandatory, especially in older children with DRE localized to the left hemisphere.
Vagus nerve stimulation (VNS)
VNS is currently FDA-approved in children 12 years and older to decrease seizure frequency. VNS is considered a second-line treatment as it is not does not produce seizure freedom but does result in a reduction of seizures. Its use is indicated in patients with DRE who are not candidates for resection or disconnection procedures. In 2011, a single-center retrospective study from New York University reviewed 141 consecutive patients who underwent VNS, 61% under the age of 12 years, with a minimum of one-year follow-up (mean, 5 years) (62). Seizure frequency was reduced by at least 50% in 64.8% of patients and was reduced by 75% in 41.4% of patients. The complication rate was 6.4%, and two-third of complications were minor. Despite the successful reduction in seizure frequency, the number of seizure medications (average 3) was not reduced (62). VNS therapy is an excellent palliative therapy in all children, including those under 12 years, who have persistent seizures after surgery or who are not candidates for focal resection with curative intent.
Corpus callosotomy
The corpus callosum, the largest white matter tract connecting the two cerebral hemispheres, allows rapid seizure propagation. Children with atonic seizures or “drop attacks” often fall, which may lead to severe brain injury and bodily harm. Atonic seizures are a frequent component of Lennox-Gastaut syndrome and other epileptic encephalopathies (63,64). Callosotomy of the anterior two-thirds or complete corpus callosum is a surgical option for children with pharmacoresistant drop seizures who have failed or are not candidates for focal resection and who have failed a trial of VNS therapy (65,66). Corpus callosotomy has also been successful in patients with recurrent or medically refractory status epilepticus (67). Preservation of the splenium, especially in older children with good language skills, may prevent a disconnection syndrome, characterized by temporary or permanent language and memory deficits.
A recent study conducted in Sweden prospectively analyzed 31 patients with DRE treated with corpus callosotomy (68). The mean age at surgery was 13.3 years, and only one patient had no clear neurologic deficit preoperatively. Twenty-five (81%) patients had two or more different seizure types, and 18 patients suffered from atonic seizures. All patients were monitored for at least two years, and 20 patients had follow-up for more than ten years. For all seizure types, nearly half (15/31) of the patients had at least a 50% reduction in seizure frequency at two years, with three patients experiencing worsening seizures. This benefit was durable, with a mean overall reduction in seizure frequency of 68% ten years after surgery. One third of the 18 patients with atonic or drop seizures experienced complete remission at two years, while two thirds of the remaining 12 patients had at least a 50% reduction. Ten years after surgery, 10/18 (56%) patients with atonic seizures had complete resolution, 6/18 (33%) had at least 50% improvement in atonic seizure frequency, and two patients were lost to follow-up (68).
Special considerations: infants
Rates of epilepsy are highest in the infant age group (69-77), with an estimated incidence of 70.1 per 100,000 (77). Furthermore, in one third of children presenting at less than 36 months of age, the epilepsy will become drug resistant (78). As surgical and anesthetic techniques continue to improve, surgery for DRE in infants has become safer. Thus, given the natural history of DRE with seizure onset in infancy (7), early surgical intervention is preferred, including relatively large resections or disconnections when indicated. In addition to seizure remission, it may be possible to avoid the financial burden and potentially harmful side effects of antiepileptic medications during early brain development (79-84). Early surgery also takes advantage of increased neuroplasticity in infants, which rapidly decreases with age.
A recent prospective cohort study of 47 children with a history of DRE who had epilepsy surgery before the age of four analyzed clinical outcomes at 2 and 10 years after surgery; 68% of patients had preoperative neurodevelopmental impairment. The mean age of seizure onset was 15 months, and the mean frequency was 150 seizures per month. The mean age at surgery was 25 months. The most common operations included frontal lobectomy, temporal lobectomy, and hemispherectomy (12 each); eight of the 47 patients ultimately required additional epilepsy surgery. The most common pathological substrates were malformations of cortical development. The complication rate was low: one child had an epidural abscess, one patient had pneumonia, and two children who underwent hemispherectomy required cerebrospinal fluid diversion with a shunt. Seventy percent of children (33/47) experienced a decrease of at least 75% in seizure frequency, and 21 (45%) achieved complete remission. Four children had worsening seizures. The best outcomes were seen in patients who underwent temporal lobectomy or hemispherectomy. In long-term follow-up, two children achieved late remission and four had late seizure recurrence (85).
Conclusions
DRE is a significant public health issue, with extremely high direct and indirect costs. Early age of seizure onset and DRE are major risk factors for poor cognitive development (7). Children with DRE face barriers to social integration and living independently, including driving and employment. Dedicated multidisciplinary teams of pediatric subspecialists are necessary to evaluate and treat these patients early in the course of their disease to optimize outcome. Seizure localization is important because it offers the best opportunity for complete lesion removal and seizure freedom. In patients in whom noninvasive methods of seizure localization fail to indicate a seizure focus, invasive methods can provide more detailed information. Once an epileptic focus is identified, resection or disconnection of the lesion from normal brain structures may help achieve the ultimate goal of complete seizure remission.
Acknowledgements
We thank Kristin Kraus, MSc, for her critical editorial assistance with this paper.
Footnote
Conflicts of Interest: The authors have no conflicts of interest to declare.
References
- Centers for Disease Control and Prevention. Epilepsy in adults and access to care—United States, 2010. MMWR Morb Mortal Wkly Rep 2012;61:909-13. [PubMed]
- Russ SA, Larson K, Halfon N. A national profile of childhood epilepsy and seizure disorder. Pediatrics 2012;129:256-64. [Crossref] [PubMed]
- U.S. Census Bureau. Annual estimates of the resident population by sex, age, race, and Hispanic origin for the United States, States, and Counties: April 1, 2010, to July 1, 2013 [database on the Internet]. 2014 [Accessed February 2, 2015]. Available online: https://www.census.gov/popest/data/counties/asrh/2012/PEPSR6H.html
- England MJ, Liverman CT, Schultz AM, et al. Epilepsy across the spectrum: promoting health and understanding. A summary of the Institute of Medicine report. Epilepsy Behav 2012;25:266-76. [Crossref] [PubMed]
- Schuele SU, Luders HO. Intractable epilepsy: management and therapeutic alternatives. Lancet Neurol 2008;7:514-24. [Crossref] [PubMed]
- Kwan P, Arzimanoglou A, Berg AT, et al. Definition of drug resistant epilepsy: consensus proposal by the ad hoc Task Force of the ILAE Commission on Therapeutic Strategies. Epilepsia 2010;51:1069-77. [Crossref] [PubMed]
- Berg AT, Zelko FA, Levy SR, et al. Age at onset of epilepsy, pharmacoresistance, and cognitive outcomes: a prospective cohort study. Neurology 2012;79:1384-91. [Crossref] [PubMed]
- Kossoff EH, Zupec-Kania BA, Amark PE, et al. Optimal clinical management of children receiving the ketogenic diet: recommendations of the International Ketogenic Diet Study Group. Epilepsia 2009;50:304-17. [Crossref] [PubMed]
- Klepper J. GLUT1 deficiency syndrome in clinical practice. Epilepsy Res 2012;100:272-7. [Crossref] [PubMed]
- Cross JH, Jayakar P, Nordli D, et al. Proposed criteria for referral and evaluation of children for epilepsy surgery: recommendations of the Subcommission for Pediatric Epilepsy Surgery. Epilepsia 2006;47:952-9. [Crossref] [PubMed]
- Dorfmüller G, Ferrand-Sorbets S, Fohlen M, et al. Outcome of surgery in children with focal cortical dysplasia younger than 5 years explored by stereo-electroencephalography. Childs Nerv Syst 2014;30:1875-83. [Crossref] [PubMed]
- Bilginer B, Yalnizoglu D, Soylemezoglu F, et al. Surgery for epilepsy in children with dysembryoplastic neuroepithelial tumor: clinical spectrum, seizure outcome, neuroradiology, and pathology. Childs Nerv Syst 2009;25:485-91. [Crossref] [PubMed]
- Giulioni M, Rubboli G, Marucci G, et al. Seizure outcome of epilepsy surgery in focal epilepsies associated with temporomesial glioneuronal tumors: lesionectomy compared with tailored resection. J Neurosurg 2009;111:1275-82. [Crossref] [PubMed]
- Engel J Jr, Kuhl DE, Phelps ME, et al. Comparative localization of epileptic foci in partial epilepsy by PCT and EEG. Ann Neurol 1982;12:529-37. [Crossref] [PubMed]
- Pondal-Sordo M, Diosy D, Tellez-Zenteno JF, et al. Usefulness of intracranial EEG in the decision process for epilepsy surgery. Epilepsy Res 2007;74:176-82. [Crossref] [PubMed]
- Wyllie E, Lachhwani DK, Gupta A, et al. Successful surgery for epilepsy due to early brain lesions despite generalized EEG findings. Neurology 2007;69:389-97. [Crossref] [PubMed]
- Wyllie E, Luders H, Morris HH, et al. The lateralizing significance of versive head and eye movements during epileptic seizures. Neurology 1986;36:606-11. [Crossref] [PubMed]
- Korff CM, Nordli DR Jr. The clinical-electrographic expression of infantile seizures. Epilepsy Res 2006;70 Suppl 1:S116-31. [Crossref] [PubMed]
- Wilmshurst JM, Gaillard WD, Vinayan KP, et al. Summary of recommendations for the management of infantile seizures: Task Force Report for the ILAE Commission of Pediatrics. Epilepsia 2015;56:1185-97. [Crossref] [PubMed]
- Barkovich AJ, Kjos BO, Jackson DE Jr, et al. Normal maturation of the neonatal and infant brain: MR imaging at 1.5 T. Radiology 1988;166:173-80. [Crossref] [PubMed]
- Altman NR, Bernal B. Pediatric applications of functional magnetic resonance imaging. Pediatr Radiol 2015;45 Suppl 3:S382-96. [Crossref] [PubMed]
- Bookheimer SY. Functional MRI applications in clinical epilepsy. Neuroimage 1996;4:S139-46. [Crossref] [PubMed]
- Nagel BJ, Barlett VC, Schweinsburg AD, et al. Neuropsychological predictors of BOLD response during a spatial working memory task in adolescents: what can performance tell us about fMRI response patterns? J Clin Exp Neuropsychol 2005;27:823-39. [Crossref] [PubMed]
- Lazeyras F, Blanke O, Perrig S, et al. EEG-triggered functional MRI in patients with pharmacoresistant epilepsy. J Magn Reson Imaging 2000;12:177-85. [Crossref] [PubMed]
- Jayakar P, Gaillard WD, Tripathi M, et al. Diagnostic test utilization in evaluation for resective epilepsy surgery in children. Epilepsia 2014;55:507-18. [Crossref] [PubMed]
- Heers M, Rampp S, Stefan H, et al. MEG-based identification of the epileptogenic zone in occult peri-insular epilepsy. Seizure 2012;21:128-33. [Crossref] [PubMed]
- Mohamed IS, Gibbs SA, Robert M, et al. The utility of magnetoencephalography in the presurgical evaluation of refractory insular epilepsy. Epilepsia 2013;54:1950-9. [Crossref] [PubMed]
- Park HM, Nakasato N, Tominaga T. Localization of abnormal discharges causing insular epilepsy by magnetoencephalography. Tohoku J Exp Med 2012;226:207-11. [Crossref] [PubMed]
- Iwasaki M, Pestana E, Burgess RC, et al. Detection of epileptiform activity by human interpreters: blinded comparison between electroencephalography and magnetoencephalography. Epilepsia 2005;46:59-68. [Crossref] [PubMed]
- Knake S, Halgren E, Shiraishi H, et al. The value of multichannel MEG and EEG in the presurgical evaluation of 70 epilepsy patients. Epilepsy Res 2006;69:80-6. [Crossref] [PubMed]
- Heers M, Rampp S, Kaltenhauser M, et al. Detection of epileptic spikes by magnetoencephalography and electroencephalography after sleep deprivation. Seizure 2010;19:397-403. [Crossref] [PubMed]
- Agirre-Arrizubieta Z, Huiskamp GJ, Ferrier CH, et al. Interictal magnetoencephalography and the irritative zone in the electrocorticogram. Brain 2009;132:3060-71. [Crossref] [PubMed]
- Huiskamp G, Agirre-Arrizubieta Z, Leijten F. Regional differences in the sensitivity of MEG for interictal spikes in epilepsy. Brain Topogr 2010;23:159-64. [Crossref] [PubMed]
- Heers M, Hirschmann J, Jacobs J, et al. Frequency domain beamforming of magnetoencephalographic beta band activity in epilepsy patients with focal cortical dysplasia. Epilepsy Res 2014;108:1195-203. [Crossref] [PubMed]
- Kaiboriboon K, Nagarajan S, Mantle M, et al. Interictal MEG/MSI in intractable mesial temporal lobe epilepsy: spike yield and characterization. Clin Neurophysiol 2010;121:325-31. [Crossref] [PubMed]
- Kharkar S, Knowlton R. Magnetoencephalography in the presurgical evaluation of epilepsy. Epilepsy Behav 2015;46:19-26. [Crossref] [PubMed]
- Cornford EM, Gee MN, Swartz BE, et al. Dynamic [18F]fluorodeoxyglucose positron emission tomography and hypometabolic zones in seizures: reduced capillary influx. Ann Neurol 1998;43:801-8. [Crossref] [PubMed]
- Foldvary N, Lee N, Hanson MW, et al. Correlation of hippocampal neuronal density and FDG-PET in mesial temporal lobe epilepsy. Epilepsia 1999;40:26-9. [Crossref] [PubMed]
- Goffin K, Dedeurwaerdere S, Van Laere K, et al. Neuronuclear assessment of patients with epilepsy. Semin Nucl Med 2008;38:227-39. [Crossref] [PubMed]
- Koepp MJ, Woermann FG. Imaging structure and function in refractory focal epilepsy. Lancet Neurol 2005;4:42-53. [Crossref] [PubMed]
- Newberg AB, Alavi A. PET in seizure disorders. Radiol Clin North Am 2005;43:79-92. [Crossref] [PubMed]
- Chandra PS, Vaghania G, Bal CS, et al. Role of concordance between ictal-subtracted SPECT and PET in predicting long-term outcomes after epilepsy surgery. Epilepsy Res 2014;108:1782-9. [Crossref] [PubMed]
- Krsek P, Kudr M, Jahodova A, et al. Localizing value of ictal SPECT is comparable to MRI and EEG in children with focal cortical dysplasia. Epilepsia 2013;54:351-8. [Crossref] [PubMed]
- Roth J, Olasunkanmi A, Ma TS, et al. Epilepsy control following intracranial monitoring without resection in young children. Epilepsia 2012;53:334-41. [Crossref] [PubMed]
- Kassiri J, Pugh J, Carline S, et al. Depth electrodes in pediatric epilepsy surgery. Can J Neurol Sci 2013;40:48-55. [Crossref] [PubMed]
- Bollo RJ, Carlson C, Schevon C, et al. Extraoperative functional mapping and staged resection of supratentorial tumors near eloquent cortex in children. Pediatr Neurosurg 2009;45:175-80. [Crossref] [PubMed]
- Brna P, Duchowny M, Resnick T, et al. The diagnostic utility of intracranial EEG monitoring for epilepsy surgery in children. Epilepsia 2015;56:1065-70. [Crossref] [PubMed]
- Davies KG, Phillips BL, Hermann BP. MRI confirmation of accuracy of freehand placement of mesial temporal lobe depth electrodes in the investigation of intractable epilepsy. Br J Neurosurg 1996;10:175-8. [Crossref] [PubMed]
- Bingaman WE, Bulacio J. Placement of subdural grids in pediatric patients: technique and results. Childs Nerv Syst 2014;30:1897-904. [Crossref] [PubMed]
- Yang PF, Zhang HJ, Pei JS, et al. Intracranial electroencephalography with subdural and/or depth electrodes in children with epilepsy: techniques, complications, and outcomes. Epilepsy Res 2014;108:1662-70. [Crossref] [PubMed]
- Roth J, Carlson C, Devinsky O, et al. Safety of staged epilepsy surgery in children. Neurosurgery 2014;74:154-62. [Crossref] [PubMed]
- Krsek P, Maton B, Korman B, et al. Different features of histopathological subtypes of pediatric focal cortical dysplasia. Ann Neurol 2008;63:758-69. [Crossref] [PubMed]
- Krsek P, Pieper T, Karlmeier A, et al. Different presurgical characteristics and seizure outcomes in children with focal cortical dysplasia type I or II. Epilepsia 2009;50:125-37. [Crossref] [PubMed]
- Lerner JT, Salamon N, Hauptman JS, et al. Assessment and surgical outcomes for mild type I and severe type II cortical dysplasia: a critical review and the UCLA experience. Epilepsia 2009;50:1310-35. [Crossref] [PubMed]
- Tassi L, Garbelli R, Colombo N, et al. Type I focal cortical dysplasia: surgical outcome is related to histopathology. Epileptic Disord 2010;12:181-91. [PubMed]
- Wilfong AA, Curry DJ. Hypothalamic hamartomas: optimal approach to clinical evaluation and diagnosis. Epilepsia 2013;54 Suppl 9:109-14. [Crossref] [PubMed]
- Fontana E, Negrini F, Francione S, et al. Temporal lobe epilepsy in children: electroclinical study of 77 cases. Epilepsia 2006;47 Suppl 5:26-30. [Crossref] [PubMed]
- Monge-Galindo L, Perez-Delgado R, Lopez-Pison J, et al. Rev Neurol 2010;50:341-8. [Mesial temporal sclerosis in paediatrics: its clinical spectrum. Our experience gained over a 19-year period]. [PubMed]
- Cersósimo R, Flesler S, Bartuluchi M, et al. Mesial temporal lobe epilepsy with hippocampal sclerosis: study of 42 children. Seizure 2011;20:131-7. [Crossref] [PubMed]
- Giordano F, Spacca B, Barba C, et al. Vertical extraventricular functional hemispherotomy: a new variant for hemispheric disconnection. Technical notes and results in three patients. Childs Nerv Syst 2015;31:2151-60. [Crossref] [PubMed]
- Hu WH, Zhang C, Zhang K, et al. Hemispheric surgery for refractory epilepsy: a systematic review and meta-analysis with emphasis on seizure predictors and outcomes. J Neurosurg 2016;124:952-61. [Crossref] [PubMed]
- Elliott RE, Rodgers SD, Bassani L, et al. Vagus nerve stimulation for children with treatment-resistant epilepsy: a consecutive series of 141 cases. J Neurosurg Pediatr 2011;7:491-500. [PubMed]
- Kim DS, Yang KH, Kim TG, et al. The surgical effect of callosotomy in the treatment of intractable seizure. Yonsei Med J 2004;45:233-40. [Crossref] [PubMed]
- Asadi-Pooya AA, Sharan A, Nei M, et al. Corpus callosotomy. Epilepsy Behav 2008;13:271-8. [Crossref] [PubMed]
- Arya R, Greiner HM, Horn PS, et al. Corpus callosotomy for childhood-onset drug-resistant epilepsy unresponsive to vagus nerve stimulation. Pediatr Neurol 2014;51:800-5. [Crossref] [PubMed]
- Rolston JD, Englot DJ, Wang DD, et al. Corpus callosotomy versus vagus nerve stimulation for atonic seizures and drop attacks: A systematic review. Epilepsy Behav 2015;51:13-7. [Crossref] [PubMed]
- Maehara T, Shimizu H. Surgical outcome of corpus callosotomy in patients with drop attacks. Epilepsia 2001;42:67-71. [Crossref] [PubMed]
- Stigsdotter-Broman L, Olsson I, Flink R, et al. Long-term follow-up after callosotomy—a prospective, population based, observational study. Epilepsia 2014;55:316-21. [Crossref] [PubMed]
- Doose H, Sitepu B. Childhood epilepsy in a German city. Neuropediatrics 1983;14:220-4. [Crossref] [PubMed]
- Granieri E, Rosati G, Tola R, et al. A descriptive study of epilepsy in the district of Copparo, Italy, 1964-1978. Epilepsia 1983;24:502-14. [Crossref] [PubMed]
- Verity CM, Ross EM, Golding J. Epilepsy in the first 10 years of life: findings of the child health and education study. BMJ 1992;305:857-61. [Crossref] [PubMed]
- Camfield CS, Camfield PR, Gordon K, et al. Incidence of epilepsy in childhood and adolescence: a population-based study in Nova Scotia from 1977 to 1985. Epilepsia 1996;37:19-23. [Crossref] [PubMed]
- Kurtz Z, Tookey P, Ross E. Epilepsy in young people: 23 year follow up of the British national child development study. BMJ 1998;316:339-42. [Crossref] [PubMed]
- Freitag CM, May TW, Pfafflin M, et al. Incidence of epilepsies and epileptic syndromes in children and adolescents: a population-based prospective study in Germany. Epilepsia 2001;42:979-85. [Crossref] [PubMed]
- Olafsson E, Ludvigsson P, Gudmundsson G, et al. Incidence of unprovoked seizures and epilepsy in Iceland and assessment of the epilepsy syndrome classification: a prospective study. Lancet Neurol 2005;4:627-34. [Crossref] [PubMed]
- Durá-Travé T, Yoldi-Petri ME, Gallinas-Victoriano F. Incidence of epilepsies and epileptic syndromes among children in Navarre, Spain: 2002 through 2005. J Child Neurol 2008;23:878-82. [Crossref] [PubMed]
- Eltze CM, Chong WK, Cox T, et al. A population-based study of newly diagnosed epilepsy in infants. Epilepsia 2013;54:437-45. [Crossref] [PubMed]
- Wirrell E, Wong-Kisiel L, Mandrekar J, et al. Predictors and course of medically intractable epilepsy in young children presenting before 36 months of age: a retrospective, population-based study. Epilepsia 2012;53:1563-9. [Crossref] [PubMed]
- Jonas R, Nguyen S, Hu B, et al. Cerebral hemispherectomy: hospital course, seizure, developmental, language, and motor outcomes. Neurology 2004;62:1712-21. [Crossref] [PubMed]
- Jonas R, Asarnow RF, LoPresti C, et al. Surgery for symptomatic infant-onset epileptic encephalopathy with and without infantile spasms. Neurology 2005;64:746-50. [Crossref] [PubMed]
- Freitag H, Tuxhorn I. Cognitive function in preschool children after epilepsy surgery: rationale for early intervention. Epilepsia 2005;46:561-7. [Crossref] [PubMed]
- Loddenkemper T, Holland KD, Stanford LD, et al. Developmental outcome after epilepsy surgery in infancy. Pediatrics 2007;119:930-5. [Crossref] [PubMed]
- Skirrow C, Cross JH, Cormack F, et al. Long-term intellectual outcome after temporal lobe surgery in childhood. Neurology 2011;76:1330-7. [Crossref] [PubMed]
- Viggedal G, Olsson I, Carlsson G, et al. Intelligence two years after epilepsy surgery in children. Epilepsy Behav 2013;29:565-70. [Crossref] [PubMed]
- Reinholdson J, Olsson I, Edelvik A, et al. Long-term follow-up after epilepsy surgery in infancy and early childhood--a prospective population based observational study. Seizure 2015;30:83-9. [Crossref] [PubMed]