Innovations in cell therapy in pediatric diseases: a narrative review
Introduction
Stem cells are undifferentiated cells with the capability to proliferate (self-renewal) and differentiate into various mature cell types (multipotent) (1). In optimal ex-vivo conditions, stem cells can be stimulated to regenerate and differentiate into specific cell types (2). There are multiple sources of stem cells, including but not limited to the bone marrow (3), peripheral blood (4), adipose tissue (5), and umbilical cord blood (6).
Stem cell therapy is a regenerative medicine modality that has the potential to decrease morbidity and mortality by promoting tissue regeneration and/or modulating the inflammatory response. Stem cell-based therapy can be autologous, where the patient’s cells are used, or allogenic, where cells from a healthy donor are used for treatment (7). Consequently, stem cell-based therapies have become of great interest in research and clinical therapeutic platforms for the treatment of various pediatric diseases. An increase in the number of clinical trials studying the safety, and efficacy of stem cell therapy in pediatric diseases has led to advancements in the selection of donors, cell sources, and administrative care.
In this literature review (Figure 1), we discuss the different sources of cell therapy and the wide spectrum of cell therapy trials for pediatric diseases. This review aims to inform researchers and clinicians about preclinical and clinical stem cell therapy trials in pediatrics. We discuss pre-clinical and clinical stem cell therapy studies in pediatric disorders emphasizing the outcomes, and advancements in this field. Due to the large landscape of the topic, we selected specific diseases to discuss in this review. We included non-hematologic pediatric diseases with current increased research interest, and recent research advancements in cell therapy applications. The review will mainly discuss and focus on mesenchymal stem cells (MSCs), bone marrow mononuclear cells (MNCs), multipotent adult progenitor cells (MAPCs), neural stem cells (NSCs), and umbilical cord blood cells (UCBC) preclinic and clinical applications in pediatric diseases. This review will cover pediatric neurological, musculo-skeletal, pulmonary, cardiac and graft-vs.-host diseases (GvHDs). For each specific diseases mentioned, we will discuss pre-clinical and clinical research according to the type of cell stem studied. We also highlight the different stem cells sources, methods of administration, and therapeutic approaches relative to each disease. We present this article in accordance with the Narrative Review reporting checklist (available at https://tp.amegroups.com/article/view/10.21037/tp-23-92/rc).
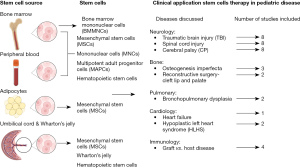
Methods (Table 1)
Table 1
Items | Specification |
---|---|
Date of search | 28/10/2022 |
Databases and other sources searched | PubMed, ClinicalTrials.gov |
Search terms used | “Stem cell” or “stem cell therapy” |
Timeframe | 2000–2022 |
Inclusion and exclusion criteria | Inclusion: pre-clinical studies, animal model studies, Clinical trials, multicenter studies, case reports, meta-analysis and systematic reviews |
Exclusions: articles written in languages other than English; those that did not recruit patients <18 years of age; studies that did not include stem cell therapy as primary goal | |
Selection process | Authors conducted the selection independently; consensus was obtained after discussion |
PubMed database was searched on October 28, 2022, using the following Medical Subject Headings (MeSH) terms (“stem cell” or “stem cell therapy”) with an age filter (child: birth − 18 years). Our search was limited to publications published between 2000 and 2022 in English. Pre-clinical, animal model studies, clinical trials, case reports, observational studies, and systemic analyses were considered in our search. Our search resulted in 3,812 articles. Abstracts were screen in first round followed by full-text screening, 38 relevant studies were included in this review.
Additionally, we included completed clinical trials with available results from the ClinicalTrials. gov database. The search term used “stem cell” with an age filter (age 0–17 years). The search resulted in 87 clinical trials and 1 observational study. Terminated studies without results and those that did not recruit patients aged <18 years were excluded, while studies that included both pediatric and adults were included in this review. Studies conducted after stem cell transplantation or treatment that did not include stem cells as the primary goal were excluded from this review.
Our review was limited to specific pre-selected diseases discussed below. Diseases were selected after a first holistic review of the yielded PubMed search according to increase research interest and advancements in the field. Our search strategy is limited to used MeSH terms additionally “stem cell”, which may have limited and excluded studies that referred to MSCs as stromal cells.
Cell sources (Figure 1)
MSCs
MSCs are stromal cells with multilineage differentiation properties with the ability to differentiate under stimuli factors into various tissues including osteoblasts, chondrocytes, hepatocytes, and epithelial cells (8). MSCs can be extracted from numerous tissues, most commonly from the bone marrow (9), adipose tissue (5), and umbilical cord (6). MSCs have shown high immunoregulatory potential due to their ability to secrete interleukin (IL)-10, transforming growth factor (TGF)-β1, prostaglandin E2 (PGE2), and indoleamine 2,3-dioxygenase (IDO), in addition to inhibiting pro-inflammatory cytokines tumor necrosis factor (TNF)-α, interferon (IFN)-γ, and IL-12. These cytokines play an important role in regulating the immune system and T-cell response. MSCs regulate T helper (Th)1/Th17 by stimulating T-regulatory and Th2 cell expansion while suppressing Th17 cell proliferation and differentiation (8). MSC-derived extracellular vesicles (EVs) have been a point of interest as a cell-free therapy in regenerative medicine. EVs contain “cytokines and growth factors, signaling lipids, mRNAs, and regulatory miRNAs” that can contribute to inflammatory regulation and tissue regeneration (10-12).
MSCs were first used as cell therapy for the treatment of GvHD, since then MSCs cell therapy has been implemented in various inflammatory diseases such as sepsis, chronic inflammatory central nervous system (CNS) injuries, and bronchopulmonary dysplasia (BPD). MSC therapy is considered safe and feasible. A meta-analysis of randomized clinical trials found no link between MSC and acute infusion toxicity, infection, mortality, or risk for tumor formation (13). However, there was a substantial link between MSC and transitory fever after up to 90 months of follow-up. Currently, 381 clinical trials studying MSCs’ therapeutic potential and side effects in various disorders are registered on clinicaltirals.gov; of these 381 studies, only 61 targeted the pediatric population.
Although it was previously thought that MSCs migrate and home to the site of injury, various research groups have debunked this concept. In several in vivo studies, MSCs were trapped in the lungs following intravenous injection (14). No viable MSC were detected in other organs, including the liver, spleen, kidneys, CNS, and bone marrow (15). MSCs have been shown to improve functional recovery after traumatic brain injury (TBI) without homing in the brain parenchyma. In an animal model, 48 hours post-MSC infusion, cells were localized to the lung, and only 0.0005% of injected cells reached the cerebral parenchyma (16).
BMMNCs
In the mononuclear portion of normal human adult BM, numerous cells of nonhematopoietic lineage or that can develop into nonhematopoietic cells have been identified (17). MNCs are a combination of several types of cells that include hematopoietic progenitors, lymphoid cells, and monocytes. Bone marrow-derived MNCs (BMMNCs) produce significant amounts of anti-inflammatory cytokines and growth factors at the tissue level. Cytokines and growth factors produced inhibit apoptosis, protect viable cells, decrease fibrosis, stimulate cell recruitment, and have anti-inflammatory properties. Moreover, BMMNCs have been shown to stimulate angiogenesis and tissue regeneration by mediating resident progenitor cells (18).
CD133+ cells, a subset of BMMNCs, have been proven in vivo and in vitro to be capable of differentiating into tissues of mesodermal, ectodermal, and endodermal origin. These cells can generate multilineage hematopoiesis and regenerate cells of various organs, such as the liver, lung, gut, muscle, and endothelial cells, when transplanted into animal models (19). BMMNCs and CD133+ cell types have recently been employed in novel therapeutic applications, including the treatment of ischemic disorders and neurological diseases, such as TBI.
MAPCs
MAPCs are a sub-type of adult nonhematopoietic stem cells that can be isolated from bone, or muscle tissue (20). MAPCs have a different phenotype and differentiation potential than MSCs and are characterized by extended expansion potential with higher and more stable telomerase activity. MAPCs can differentiate in vitro and in vivo into various cell types including osteoblasts, chondrocytes, smooth muscle cells, and endothelial-like cells. Compared with MSCs, MAPCs express lower levels of major histocompatibility complex (MHC) class I (21). In a murine model isolated MAPCs inhibited T-cell proliferation and activation via elaboration of PGE2 (22). Additionally, human MAPCs increased angiogenesis and stem cell proliferation when injected into the CNS and cardiac in vivo ischemic models (23). Due to their unique properties, MAPCs have been studied in multiple clinical trials as a source of cell therapy treatment for graft vs. host, TBI, and cardiovascular diseases.
NSCs
NSCs are primordial cells of the nervous system that can differentiate into more specialized cells of all neural lineages in the central and peripheral nervous system including neurons, astrocytes, and oligodendrocytes (24). The subventricular zone (SVZ) is considered the richest source of NSCs, which later on proliferate to neuroblasts cells. Neuroblasts migrate to the olfactory bulb and differentiate into subtypes of interneurons (25). Intraventricular transplantation of NPCs in murine experimental autoimmune encephalomyelitis (EAE) models decrease clinical disease severity and neuroinflammation (26). Further investigation confirmed the immunomodulatory properties of NSCs (27). Neuroprotective effect was also observed in studies after treatment with NSCs, through reducing parenchymal necrosis and glial scar formation (28,29).
UCBCs
The umbilical cord is considered a waste material, but it is a highly valuable source of hematopoietic stem cells and MSCs. Umbilical cord blood was first used as a source for hematopoietic stem cells in the treatment of hematologic disorders. An increase in the utilization of allogeneic umbilical cord blood stem cells transplant is due to the quick availability of stem cells, where umbilical cord stem cells were transplanted 25–36 days earlier compared to bone marrow stem cell transplantation (30,31). Additionally, 1 or 2 human leukocyte antigen (HLA) (HLA-A, B, and DR) antigens mismatch between UBC stem cells and donor is tolerated (30). The less strict requirements for HLA matching increase utilization as an alternative source of hematopoietic cells for transplantation (32). Multiple studies confirmed that umbilical blood transplant is associated with a lower incidence of GvHD in children compared to bone marrow transplant (33,34). The use of UCB stem cells is emerging in regenerative medicine and immunomodulation therapies. UCB MSCs have been implemented in various clinical trials studying the efficacy and safety of UCB stem cell therapy including in CNS trauma, cerebral palsy (CP), and osteogenesis imperfecta (OI). The availability, high tolerance to HLA mismatch, and decreased GvHD are all advantages of employing cord blood in stem cell therapy.
Wharton’s jelly (WJ)
WJ is a jelly-like substance within umbilical cords that surrounds the umbilical arteries and veins. It is made of a collagen extracellular matrix (ECM) with glycoprotein microfibrils embedded with soluble polysaccharides, hyaluronan (HA), and stem cells (35). HA plays a role in many biological processes, such as angiogenesis and proliferation/differentiation of progenitor cells. In addition, collagen matrix has a high compatibility for supporting many cell types. Growth factors [insulin-like growth factor binding protein (IGFBP), TGF-α], immunomodulatory, anti-inflammatory cytokines, homeostatic cytokines, and cytokines associated with wound healing were detected in WJ samples. WJ was found to have higher amounts of these factors compared with other biologics making its great therapeutic potential in regenerative medicine and reducing inflammation (36).
Cardiosphere-derived cells (CDCs)
CDCs are cardiac progenitor cells that can differentiate into three cell types: cardiomyocytes, smooth muscle cells, and endothelial cells (37). CDCs are obtained from percutaneous endomyocardial biopsy and then grown in-vitro to generate functioning CDCs (38). A study by Davis et al. has shown the regenerative capabilities of CDCs in mice models (39). There have been four completed phase I trials incorporating CDCs in patients with promising results.
Stem cell therapies (Table 2)
Table 2
Disease | Citation | Trial | Characteristics | Stem cell | Outcomes |
---|---|---|---|---|---|
TBI | (40) | Phase 1 clinical trial | Ten children aged 5 to 14 years; Glasgow coma scale of 5 to 8 | Autologous BMMNCs | Improvement on the Glasgow coma scale |
Neuroprotection | |||||
(41,42) | Animal model | Rats, controlled cortical impact injury | MAPCs | Increase in brain macrophage M2 phenotype | |
Improved BBB permeability and spatial learning | |||||
(43,44) | Animal model | Rats, controlled cortical impact injury | MSCs | Attenuation of TBI-induced inflammatory responses | |
(45,46) | Animal model | Rat/mouse TBI model | MSC exosomes | Reduced neuro-inflammation | |
Improved functional recovery | |||||
Promoted microglia transformation to anti-inflammatory phenotype | |||||
(47) | In vitro and in vivo animal models | Male Sprague-Dawley rats | Umbilical cord Treg+ MSC | Attenuation of central and peripheral immune response | |
Decreased chronic microgliosis | |||||
SCI | (48,49) | Clinical trial (open label) | Pediatric patients age <18 years with documented SCI | Autologous BMMNC | Varying degrees of improvement in muscular power, bladder control, balance, and sensation |
(50) | Animal model | Rate SCI model | MSCs | Improved locomotor function and caused spinal cord lesions reduction | |
(51) | Phase 1/2 clinical trial | Complete or incomplete trauma-induced SCI | Allogeneic hUC MSCs | Improvement of pinprick, light touch, motor, and sphincter scores | |
(52,53) | Animal model | Rat SCI model | MSC-derived EV | Diminished the inflammatory response | |
Improvement in locomotor recovery and mechanical sensitivity threshold | |||||
Suppressed A1 neurotoxic astrocyte activation and nitric oxide release in microglia | |||||
(54,55) | Phase 1/2 clinical trial | – | NSCs and olfactory ensheathing cells | Improvement in ASIA motor and sensory scores | |
Improvements in walking index for SCI and functional independence measure | |||||
CP | (56) | Non-randomized, observer-blinded controlled clinical | – | Autologous neuronal stem cell-like cells | Improved GMFM scores by 58% |
(57) | Randomized, blinded, placebo controlled, crossover clinical trial | – | BMMNCs and autologous UCBCs | Improvements in myelination and modest improvements in GMFM scales | |
Decrease in corticospinal tract radial diffusivity | |||||
Connectivity strength improved in either or both pathways (corticio-striatal and thalamo-cortical) | |||||
(58) | Double-blind, placebo controlled, crossover trial | – | Autologous cord blood | Improved GMFM-66 scores by a median of 3.6 points greater than expected | |
Improvement in the Peabody Developmental Motor Scales-2 Gross Motor Quotient scores | |||||
Increased normalized whole-brain connectivity compared with subjects receiving lower doses | |||||
(59) | Phase 1 open-label clinical trial | – | HLA-matched or partially HLA-matched sibling umbilical cord blood therapy | Transfusion did not elicit acute infusion reactions | |
Mean ± SD increase of 4.7±2.5 points on the GMFM-66 and 1.0±2.9 points on the Peabody Gross Motor Quotient | |||||
(60,61) | Randomized double-blind sham-controlled clinical trial | Spastic CP aged 4–14 years old | Umbilical cord blood MSC | Significant increase in mean GMFM-66 score and PEDI score | |
Improvements in ADL, CFA and GMFM | |||||
(62,63) | Animal model | HI in immunodeficient mice | Human UCBCs | Improved neurobehavioral status and alleviated pathological brain injury | |
Rat model of neonatal hypoxia/ischemia | Temporarily increase microglia in the periventricular striatum, protect neurons in the neocortex | ||||
OI | (64) | Case series | – | Bone marrow MSCs | Reduced number of fracture and improvement in quality of life |
Increased bone mineral density | |||||
(65) | Clinical report | – | Matched whole bone marrow transplantation | Decrease frequency of fracture by 80% | |
(66) | Human clinical trial | – | Pre- and postnatal transplantation of fetal MSCs | Improved linear growth, mobility, and a decrease in fracture | |
CLP | (35) | Animal rat model | Male Sprague-Dawley rats (300–320 g) | WJ | New bone growth resulting in partial to full closure of the alveolar defect |
(67) | Animal model | Female Wistar rats weighing 320 to 420 g | DPSCs | Equivalent regeneration for repairing maxillary alveolar defects between DPSC transplantation and iliac bone grafts | |
BPD | (68,69) | Phase 1/2 clinical trial | – | MSCs | Progressive decrease in parenchymal lung fibrosis |
Reduction in inflammatory cytokines | |||||
Heart failure | (70) | Phase 1/2 clinical trial | – | BMMNCs | LV volumes were reduced, significant differences in EDV and ESV |
HLHS | (71) | Phase I/IIb clinical trial | – | MSC | Cell therapy was well tolerated, no adverse outcomes |
Ongoing phase II | |||||
(72) | Non-randomized, prospective controlled study | – | CDCs | Significantly increased RVEF | |
GvHD | (73) | Human clinical trials meta-analysis | aGvHD | MSC | 205 of 301 patients exhibited ORR |
Children tend to have better clinical responses than adults | |||||
Pure skin involvement showed higher clinical efficacy | |||||
(74) | Case report | aGvHD | MSC | Effectively and safely treated steroid-resistant gastrointestinal GvHD | |
(75) | Phase 1/2 clinical trial | Chronic GvHD | MSC | Patients were off steroids −80% were in complete remission | |
(76) | Case report | Chronic GvHD | MSC | Treated steroid refractory GvHD patient with nephrotic syndrome | |
24-h urinary protein and serum albumin levels returned to normal |
TBI, traumatic brain injury; BMMNC, bone marrow-derived MNC; MNC, mononuclear cell; MAPC, multipotent adult progenitor cell; BBB, blood-brain barrier; MSC, mesenchymal stem cell; Treg, regulatory T cells; SCI, spinal cord injury; hUC, human umbilical cord; EV, extracellular vesicle; NSC, neural stem cell; ASIA, American Spinal Injury Association; CP, cerebral palsy; GMFM, Gross Motor Function Measurement; UCBC, umbilical cord blood cell; HLA, human leukocyte antigen; SD, standard deviation; PEDI, Pediatric Evaluation of Disability Inventory; ADL, Activity of Daily Living; CFA, Comprehensive Function Assessment; OI, osteogenesis imperfecta; CLP, cleft lip and palate; WJ, Wharton’s jelly; DPSC, dental pulp stem cell; BPD, bronchopulmonary dysplasia; LV, left ventricle; EDV, end-diastolic volume; ESV, end-systolic volume; HLHS, hypoplastic left heart syndrome; CDC, cardiosphere-derived cell; RVEF, right ventricle ejection fraction; GvHD, graft-vs.-host disease; aGvHD, acute GvHD; ORR, overall response rate.
Neurological disorders
TBI
TBI occurs due to an external insult that causes damage and inflammation in the brain. TBI is characterized by acute phase inflammation which triggers a cellular cascade of chronic secondary neuroinflammation (77). In the US, 2.87 million cases of TBI were reported in 2014, with more than 837,000 of those cases involving pediatrics. In the United States, an estimated 13.5 million people struggle with disability from TBI (78). Therapeutic stem cell therapy with BMMNCs, MAPCs (23), and MSCs (42) have shown promising results in improving outcomes and limiting secondary injury. Stem cell therapy has been shown to modulate cellular responses and inflammatory cascades in TBI (79).
BMMNCs cell therapy
A clinical trial using BMMNCs was implemented in an acute injury setting in pediatric patients with TBI. The phase 1 clinical trial included 25 patients, demonstrated that acute bone marrow harvest and infusion in an acute injury setting is safe and feasible. Additionally, cell therapy was associated with progressive gradual improvement in functional outcomes after 6 months. Moreover, upon follow up patients treated with BMMNCs did not suffer from expected post-TBI progressive CNS tissue loss. Cell therapy offered neuroprotection with 70% of patients showing improvement on the Glasgow coma scale (40).
MAPCs
MAPCs offer neuroprotection, attenuate blood-brain barrier (BBB) permeability, increased anti-inflammatory cytokine production, increase the percentage of T-regulatory cells, and preserve splenic mass after TBI (41). Intravenous administration of MAPCs in animal models increased T regulatory cell populations within the spleen and peripheral blood at 24 and 48 hours respectively. The increase in T-regulatory cells modulates the immune system, leading to an increase in the differentiation of brain microglial/macrophage cells into M2 neuroprotective phenotype (23). Recent work done by Bedi et al. studied the optimum clinically relevant time window of MAPC treatment for TBI through a series of experiments. The study found that administration of MAPCs within 24 h improved BBB permeability, spatial learning, and cognitive behavior. Additionally, it decreased activated microglia/macrophages in the dentate gyrus (42).
MSC
MSC treatment of TBI has demonstrated encouraging outcomes in both animal models and human clinical studies. MSC stem cell therapy is considered safe and feasible because no immediate or delayed toxicity linked to treatment was reported in pediatric patients at the 6-month follow-up (80). In a TBI rat model, MSCs reduced the number of microglia and other inflammatory cells, increased anti-inflammatory cytokines, and decreased proinflammatory cytokine production leading to attenuation of TBI-induced inflammatory responses (44). Furthermore, BM-MSCs injected 7 days after TBI resulted in a 50% reduction in IFN-γ and TNF-α, as well as a substantial decrease in BBB permeability, edema, and microglial activation (43).
MSC exosomes
MSC exosomes have emerged as a promising therapy for TBI. Several preclinical studies have shown that MSC exosomes can improve functional recovery, reduce brain inflammation, and promote neurogenesis in animal models of TBI. A study published in 2020 showed that treatment with MSC derived exosomes improved functional recovery, reduced inflammation, and promoted neurogenesis in a rate model of TBI (45). Another study 2022 found that MSC exosomes reduced neuroinflammation and promoted microglia transformation to anti-inflammatory phenotype in a mouse model of TBI (46). Although there is growing evidence of the therapeutic potential of MSC exosomes for TBI, there are still many challenges that need to be addressed before this therapy can be used in clinical practice. The challenge facing researchers is the development of standardized methods for the isolation, purification, and characterization of MSC exosomes. Overall, the available evidence suggests that MSC exosomes hold great promise as a therapeutic approach for TBI. However, further research is needed to optimize the isolation and characterization of MSC exosomes.
MSC therapy optimization for the treatment of TBI
Recent studies have focused on optimizing MSCs therapy potency and therapeutic applications through priming approaches, and combination cell therapy. Priming or preconditioning with cytokines, pharmacological agents, chemical agents, and hypoxia has been investigated to generate MSCs with improved potential and therapeutic efficacy (81). A paper published in 2022 introduced a novel MSCs cell therapy manufacturing approach via biomechanical conditioning without a significant impact on cell viability or identity. Reproducible results were demonstrated in adipose tissue and bone marrow human-derived MSC, with an evident increase in potency (82). Combination cell therapy to enhance therapeutic efficacy was also investigated with combination therapy with MSC + regulatory T cells (Tregs) for the treatment of TBI. Combination therapy has a higher inhibitory potency on the production of inflammatory cytokines IFN-γ and TNF-α when compared to MSC or Treg monotherapy. Additionally, in vitro rat studies have provided evidence that MSCs + Treg therapy has a higher inhibitory effect than monotherapy on the inflammatory response of microglia (47).
Spinal cord injury (SCI)
The causes of SCI are similar to TBI, where traumatic injury is the most prevalent modality. The burden of neurological damage leading to paralysis and many other debilitating systemic dysfunctions such as digestive, urogenital, and circulatory systems have increased the focus on cell therapy research for SCI (83). Similar to TBI SCI is characterized by acute injury followed by a complex cascade of secondary injury and neuroinflammation (84). Experimental studies and clinical trials investigating stem cell therapy as a therapeutic for SCI have focused mainly on the use of autologous bone marrow stem cells (85,86), MSCs (87), mesenchymal stromal cell-derived EVs (52,88), and human neural cells (89,90). Clinical trials in SCI mainly target adult populations with American Spinal Injury Association (ASIA) A with complete loss of motor and sensory function below the injury or grade B with complete motor loss (83).
BMMNCs
Autologous BMMNCs therapeutic efficacy and adverse effects were studied by various research groups. One research group assessed neurological improvement after BMMNCS therapy in pediatric patients with incurable neurological disorders and injuries, including SCI. Autologous BMMNCs were injected intramuscularly or intrathecally. At 15 months follow up, varying degrees of improvement in muscular power and bladder contractions were reported in all 4 cases of SCI enrolled in this study (48). An open-label study targeting patients with subacute and chronic SCI was conducted. The study included eleven pediatric patients, age below 18 years. BMMNCs were administered through lumbar puncture, and the patients were followed up after approximately 9 months. Patients showed symptomatic improvement in balance, trunk stability, upper limb function, mobility, sensation, and bowel/bladder functions. It was found that early intervention, multiple doses of BMMNCs, and younger patients were associated with better therapeutic outcomes (49).
MSC
MSCs have been extensively investigated as a potential therapy for SCI. Clinical trials of MSC transplantation for SCI have also shown promising results. In general, animal studies have shown that MSC therapy can improve functional recovery and reduce inflammation and tissue damage after SCI. A recent study demonstrated that infusion of human umbilical cord (hUC) derived MSCs in rat SCI model improved locomotor function and caused a dosage-dependent spinal cord lesions reduction (50).
A phase 1/2 clinical trial published in 2021 showed that subarachnoid transplantation of allogeneic hUC MSCs monthly resulted in significant and stable improvement of pinprick, light touch, motor, and sphincter scores (51). Overall, the available evidence suggests that MSCs hold great promise as a therapeutic approach for SCI. However, further research and clinical trials targeting pediatrics is needed.
Recently, researchers have been studying the therapeutic potency of MSC-derived EVs in SCI. In animal models, administration of EVs diminished the inflammatory response, with significant improvement in locomotor recovery and mechanical sensitivity threshold (52). Additionally, bone marrow MSC-derived exosomes suppressed A1 neurotoxic astrocyte activation and nitric oxide release in microglia. Animals in the treatment group were found to have a decrease in glial scar formation and neuronal apoptosis (53).
NSCs
Stem cell therapy using NSCs and olfactory ensheathing cells from the olfactory mucosa has been investigated in animal models and in human clinical trials. Olfactory mucosa autografts are considered practical and relatively safe although they are associated with surgical procedures adverse effects. Human clinical trials revealed promising results; in one study, all seven participants had improvements in ASIA motor scores, and six of those had improvements in ASIA sensory neurologic scores after autograft (54). In another clinical trial, 11 out of 20 participants showed improvements in ASIA grading, and all test participants (13 participants) had improvements in the Walking Index for Spinal Cord Injury and Functional Independence Measure (55). Since pediatric SCI patients were not the focus of these clinical studies, more research is required to determine the treatment effectiveness and potential adverse effects in this population.
CP
CP is the most common physical disability in pediatric population, with a prevalence of 3 per 1,000 life birth (91). It is caused by insult leading to abnormal development of the brain affecting movement, posture, and balance. CP is a lifelong condition associated with seizures, intellectual disabilities, and speech, vision, and hearing difficulties. Unsatisfactory outcomes with standard CP treatment approaches have pushed scientists to explore innovative therapies. In human clinical trials, stem cell treatment with umbilical cord blood MSCs and autologous neuronal stem cell-like cells yielded a spectrum of positive results.
NSC-like cells
A non-randomized, observer-blinded controlled clinical study transplanted NSC-like cells derived from autologous MSCs into the subarachnoid cavity. Compared to the baseline score, neuronal stem cell-like cell transplantation in the subarachnoid area enhanced Gross Motor Function Measurement (GMFM) scores by 58% at 6 months. Although the treatment improved gross motor function, no significant improvement in the language quotient was identified post-treatment. Because none of the 30 patients who received treatment had major ill effects or complications, neuronal stem cell-like therapy was deemed safe (56).
BMMNCs
A randomized, blinded, placebo-controlled, crossover clinical trial examined the safety and motor function improvement using an autologous mononuclear-cell-therapy-based approach to treat CP. Autologous BMMNCs or autologous umbilical cord blood were used as treatments, and total of 20 patients were randomized to 2:1 treatment:placebo. Participants who got randomized to a placebo received either BMMNCs or umbilical cord blood therapy during the 12-month post-treatment visit. The data from this study proposed that both stem cell infusions were safe. Although stem cell therapy at the doses used did not dramatically alter motor function, the data suggest that some patients may have improvements in myelination which correlates with modest improvements in GMFM scales. Ten of the 15 patients who received stem cells exhibited a consistent decrease in corticospinal tract radial diffusivity post-treatment. Furthermore, 12 months post-treatment, connectivity strength improved in either or both pathways (corticio-striatal and thalamo-cortical). This study also emphasizes the need to increase private banking or matched cord blood units to conduct larger-scale clinical studies, as such trials are not feasible at current blood bank rates (57).
UCBCs
Sun and her research group conducted a double-blind, placebo-controlled, crossover trial of autologous cord blood infusion in children with CP (58). Sixty-three eligible patients with CP aged 1–6 years were randomized to receive autologous cord blood or a placebo infusion, followed by an alternate infusion 1 year later. A dosing effect of autologous cord blood was identified, a single intravenous infusion of dose ≥2×107/kg improved GMFM-66 scores by a median of 3.6 points greater than expected. Additionally, subjects receiving high doses showed statistically significant improvement in motor functions including locomotion, posture stability, reflexes, and object manipulation. and increased normalized whole-brain connectivity compared with subjects receiving lower doses (58). The limited availability of autologous cord blood can hinder access to potentially beneficial therapies for many children with CP. A recently published open-label study conducted by the same research group showed that HLA-matched or partially HLA-matched sibling umbilical cord blood therapy is well-tolerated and potentially beneficial. The transfusion did not elicit acute infusion reactions and the reported adverse events were not related to cord blood infusions. Donor cells were not detected in the peripheral blood 6 months after infusion. Moreover, no antibody formation against platelets, red blood cells, or donor-specific HLA antigens was observed. An increase in motor assessments scales and standard scores. was reported in patients 6 months after infusion. Although the findings of the above-mentioned clinical trials are promising, more randomized clinical trials are required to assess the efficacy of cord blood therapy and to evaluate the use of allogeneic cells (59).
Clinical investigations on umbilical cord blood MSC therapy in patients with CP have revealed encouraging outcomes and improvements in gross motor skills. A randomized, double-blind, sham-controlled clinical trial assessed the safety and efficacy of intrathecal injection of umbilical cord blood MSCs in 36 patients with spastic CP aged 4–14 years old. At the 12-month follow-up, a significant increase in the mean gross motor function and pediatric evaluation of disability inventory (PEDI) scores were reported in the treatment group compared to the baseline and control groups. The modified Ashworth scale (MAS) scores, which measure resistance during passive movement, decreased after 12 months compared with the baseline and treatment groups (60). Similarly, another clinical trial administered four doses of umbilical cord-derived MSCs intravenously to investigate their safety and efficacy. The treatment arm showed significant improvements in Activity of Daily Living (ADL) assessment, Comprehensive Function Assessment (CFA), and GMFM scale (61).
A preclinical animal model study examined the effects of human UCBCs in a CP model by hypoxia-ischemic injury (HI) in immunodeficient mice over a period of 8 weeks. Both CD34+ and CD34− cells showed improved neurobehavioral status and alleviated pathological brain injury (62). Additional studies have investigated the mechanisms underlying the therapeutic effects of human UCBC therapy using a rat model of neonatal hypoxia/ischemia. Human UCBCs temporarily increase microglia in the periventricular striatum, protect neurons in the neocortex, and pave the way for near-normalization of brain damage in the SVZ (63). This research revealed that the effects of favorable behavioral functions persisted, and human UCBC therapy had a long-term positive impact through paracrine effects.
Musculoskeletal-OI
OI is a group of genetic disorders caused by mutations in the collagen type I alpha (COL1A)1 or COL1A2 genes, which encode for type 1 collagen. Most of these mutations result in an aberrant collagen microfibril assembly. The most common clinical manifestations are abnormal skeletal development, osteopenia, fracture, and short stature. Individuals with OI may also have brittle teeth, hearing loss, and hypermobile joints. There is no cure for OI, and the existing therapies do not address the underlying pathology. Given the severity of the disease and the limits of current therapies, there is a clear unmet need for OI management. Clinical trials of stem cell therapy have opened up a new avenue for treatment.
Bone marrow MSCs and whole bone marrow transplantation
MSCs are candidates for clinical therapy for OI due to their capacity to act via paracrine effects on tissues and exhibit multilineage differentiation potential with a low immunogenic profile. In a case series (64), two patients with OI were administered five IV infusions of bone marrow MSCs retrieved from their siblings over the course of 2.5 years. Assessments and follow-up during the years of therapy and at 2 years showed a reduced number of fractures and an improved quality of life. Patients’ assessments displayed increased bone mineral density that continued to increase in patients with less severe OI, even after the follow-up period. Patients with severe OI showed a decrease in some bone parameters after follow-up, which suggests that continuous treatment is necessary for the most severe patients. Another study investigated the clinical outcomes of whole bone marrow transplantation in OI patients. A decrease in the frequency of fracture by 80% on 6-month follow-up was reported in six children with OI type III after HLA-matched whole bone marrow transplantation (65).
Prenatal stem transplantation of fetal MSC
Fetuses with OI are affected by the disease even during the prenatal period. During this period, the fetus develops fractures that can be detected using ultrasound imaging. There is a drive to start therapy as soon as possible to improve clinical outcomes in fetal life or perhaps prevent fractures from occurring. Pre- and postnatal transplantation of fetal MSCs in OI results in improved linear growth, mobility, and a decrease in fracture incidence (66). At 31 weeks of gestation, a fetus with OI type IV was transplanted with fetal MSCs, resulting in no new fractures in the remainder of the pregnancy or during infancy. Postnatal infusion of MSCs, at 19 months of age, from the same donor resulted in the resumption of her developmental trajectory, which plateaued at 13 months of age after following a normal growth trajectory (92). Transplantation of allogeneic fetal MSCs in OI appears to be safe and therapeutically helpful; nevertheless, further research and studies are needed.
Birth defects-cleft lip and palate (CLP)
CLP is among the most common birth defects. CLP repair is usually performed using an autologous alveolar bone graft (ABG) from the patient’s iliac crest to fill the maxillary bone defect. However, this treatment has some disadvantages, such as delayed timing of repair, additional surgery, and donor-site complications. Novel methods to repair CLP without ABG are currently in development and have been shown to be successful in rats, namely, using WJ graft and osteoblast-differentiated human deciduous dental pulp stem cells (DPSCs).
WJ
Implantation of WJ improves bone regeneration. In a study by Sahai et al. (35), WJ was isolated from the hUC and implanted in critical-sized alveolar defects in rats. New bone growth was detected following implantation, resulting in partial to full closure of the alveolar defect compared with the controls. No ectopic bone growth was observed in this study, indicating that WJ implantation is a safe and effective treatment for enhancing bone regeneration. In addition, the newly formed bone contained extremely small amounts of human DNA, demonstrating that the regenerated bone is composed of host-derived cells.
DPSCs
DPSCs are derived from the neural crest and are a potential source of autologous stem cells in the regenerative treatment of musculoskeletal diseases (93). In the referenced study (67), osteoblast-differentiated human deciduous DPSCs were seeded onto a collagen membrane and implanted in rats with maxillary alveolar defects. On 2-month follow-up, the study reported new bone formation in the maxillary alveolar defects. Additionally, when comparing DPSC transplantation to iliac bone grafts, this study showed equivalent regeneration potential for repairing maxillary alveolar defects between DPSC transplantation and iliac bone grafts.
Pulmonary diseases-BPD
BPD is a chronic lung disease affecting premature infants. It is a developed complication of extended mechanical ventilation and supplemental oxygen therapy. Preterm infants born before 29 weeks of gestation weigh less than 1,500 g at birth, and those with respiratory distress syndrome (RDS) are at high risk of developing BPD (94). Multiple ongoing human clinical trials are currently studying the efficacy and safety of stem cell therapy, mainly MSCs, for BPD.
MSCs
Clinical trials of MSC stem cell treatment for BPD have yielded favorable results, with no major adverse effects. In a case series, four patients with BPD were administered two intravenous doses of allogeneic hUC MSCs, demonstrating the safety and efficacy of MSCs for BPD. All four patients improved and were weaned from supplemental oxygen. They also reported a progressive decrease in parenchymal lung fibrosis on imaging (68). A phase I trial administered MSCs intratracheally to high-risk preterm infants. They found a reduction in inflammatory cytokines IL-6, IL-8, matrix metalloproteinase (MMP)-9, TNF-α, and TGF-1 in tracheal aspirates of MSC-treated children compared to a case-matched control group. On the 2-year follow-up, no serious adverse effects or adverse outcomes on growth or neurodevelopment were found (69).
The optimum clinically relevant time window for MSC treatment is critical for achieving a significant improvement in BPD outcomes. A randomized controlled phase II trial found a substantial improvement in mortality and severity of BPD between those treated with MSCs and those who were not. In the subgroup study, the 23–24 gestational age group exhibited a significant improvement in severe BPD outcomes, but the 25–28 gestational age group did not (95). Thus, further clinical trials are needed to determine the optimal time window and dose of MSCs for BPD.
Cardiac diseases
Heart failure
Pediatric heart failure is rare compared to adult heart failure and is predominated by congenital malformations rather than atherosclerotic-based disease states (96). Furthermore, pediatric heart failure represents a larger burden on the healthcare system owing to the long duration of inpatient stay and lack of standardized treatment options (97). Stem cell therapy represents a potential alternative to the current surgical and transplant treatment options. Pincott et al. demonstrated the feasibility and safety of BMMNC therapy for cardiomyopathy in children. Intracoronary injection of autologous BMMNCs resulted in significant differences in end-diastolic volume (EDV) and end-systolic volume (ESV) compared with the placebo group, with an average decrease of 7% and 10%, respectively (70). Additionally, LV volumes were reduced, indicating potential cardiac reverse remodeling, which has been associated with long-term benefits in HF-related morbidities and mortalities (98).
Hypoplastic left heart syndrome (HLHS)
HLHS is a complex congenital heart disease (CHD) that accounts for 2% of all CHD (99) right ventricle (RV) dysfunction is a leading contributor to mortality (100,101). The only curative option is heart transplantation; however, this option is limited by donor availability. Cell therapy options in this field are being explored as alternatives. The ELPIS phase I trial demonstrated the efficacy of Lomcel-B injection, a preparation of MSCs, in 10 infants with HLHS after surgical intervention. Intramyocardial injection of Lomecel-B was well-tolerated with no reported adverse outcomes. The ongoing ELPIS Phase II trial (as of November 2022) plans to investigate the benefits of Lomcel-B in an expanded population (71).
CDCs
CDCs are progenitor cells that can differentiate into three cell types: cardiomyocytes, smooth muscle cells, and endothelial cells Davis et al. demonstrated the regenerative capabilities of CDCs (37,39). The TICAP trial conducted by Tarui et al. assessed the outcomes of CDC infusion in HLHS. This prospective, controlled study involved a group that received intracoronary infusion 1-month post-surgery and was evaluated through a 36-month follow-up. Compared to a control, placebo group, CDCs infusion did not result in increased complications or side effects, however, a significantly increased RV ejection fraction (RVEF) was seen, potentially ameliorating one of the major complications associated with HLHS surgical treatment (72).
GvHD
GvHD is a serious complication of allogeneic hematopoietic stem cell transplantation (HSCT), in which donor T lymphocytes attack host tissues. GvHD is typically categorized as either acute or chronic. Acute GvHD (aGvHD) occurs within 100 days and is a type IV hypersensitivity reaction (102). Chronic GvHD occurs after 100 days, and its pathophysiology is uncertain. aGvHD typically affects the skin, liver, and gastrointestinal tract, whereas chronic GvHD can involve any organ. The 2021 annual report by the Center for International Blood and Marrow Transplant Research shows that GvHD causes death in 9–16% of allogeneic unrelated matched HSCT recipients aged <18 years (103). Given that GvHD may also put HSCT patients at risk for other causes of death, such as organ failure and infection, it should be highlighted that these numbers might be underestimated.
Currently, corticosteroid therapy has an overall effectiveness rate of 50%, whereas other immunosuppressive medications have a full response rate of approximately 30% (104). For the treatment of GvHD, there is still a need for novel treatments with improved efficacy. In 2004, Le Blanc et al. published the first report on MSC therapy for GvHD, with a promising clinical response (105). Numerous studies and trials have been conducted to assess the efficacy of MSCs as a therapy for GvHD since the first case report. MSCs therapy in GvHD is thought to be safe and does not pose any significant safety concerns. Allogeneic MSCs have been used in most GvHD investigations, with bone marrow being the most frequently used tissue source (103).
MSC therapy for aGvHD
A meta-analysis focusing on the efficacy of MSC therapy for steroid-refractory aGvHD reported that 205 of 301 patients exhibited an overall response rate (ORR). In contrast to patients with gastrointestinal and liver involvement, those with pure skin involvement showed higher clinical efficacy according to the authors. Additionally, children tend to have better clinical responses than adults (73). In a recent case report, a 15-year-old patient was treated with MSC therapy for steroid-refractory gastrointestinal aGvHD. The patient received eight MSC intravenous infusions. On follow-up, diarrhea gradually subsided and laboratory test results improved. MSC therapy effectively and safely treated steroid-resistant gastrointestinal GvHD (74).
MSC therapy for chronic GvHD
MSCs have considerably reduced symptoms and complications associated with chronic GvHD. In a clinical trial, eleven patients with chronic GvHD received an infusion of MSCs in addition to the first-line treatment combination of cyclosporine and prednisone. At week 56, all patients who continued under the protocol were off steroids and 80% were in complete remission. During the clinical trial, there were no reported serious adverse effects, infection-related mortality, or relapse of the underlying disease (75). A case report published in 2017 reported complete remission after MSC therapy in a steroid-refractory GvHD patient with nephrotic syndrome. Following treatment failure with cyclosporine A, prednisone, and rituximab, the patients received six doses of allogeneic bone marrow MSCs. Two weeks after the first infusion, a rapid decrease in urinary protein levels and an improvement in edema were observed. Additionally, at follow-up, 24-h urinary protein and serum albumin levels returned to normal (76). Although many studies have presented encouraging results with MSCs therapy in the treatment of acute and chronic GvHD, the efficacy and outcomes still need to be verified by large-scale randomized controlled trials.
Methods of administration
The optimal method of administration, dose, and timing of stem cell administration vary according to the cell type and pathophysiology of the disease. Different routes of administration have been to achieve optimal results and therapeutic efficacies. Cells can be administered locally into the tissue or systematically through peripheral access. Most clinical trials employ systemic delivery, which offers easy and safe access. This method of administration promotes MSC migration to the lung microenvironment, where their positive effects are mediated by interactions with alveolar and pulmonary interstitial macrophages (14). The lung microenvironment promotes MSC secretion of factors that lead macrophages to polarize towards an immunosuppressive M2-like phenotype for tissue homeostasis restoration, which can impact MSC immune regulatory function (106). Systemically administered allogeneic stem cells may be rapidly cleared by natural killer cells and macrophages decreasing availability (107). However, MSCs and MAPCs stem cells can exert a therapeutic effect from a distance through the secretion of EVs and soluble factors with paracrine effects. Hence, the optimal route of administration is tailored to the source of stem cells and the pathophysiology of the disease.
One study focused on the effect of catheters of various sizes and flow rates on MSC viability, characterization, and function. Human and rat MSCs were characterized 24 h after injection, and no difference in surface markers or capacity for multilineage differentiation was reported when MSCs were injected through 20-, 25-, and 30-gauge needles at 60, 120, 240, and 500 mL/h flow rates. All catheters and flow rates resulted in a delayed decrease in human MSCs viability at 24 h, where MSCS viability dropped below the FDA threshold (70%) with a 20-gauge needle and SL-10 microcatheter (108).
Conclusions
Diverse sources of stem cells have different properties and mechanisms of action, which allow the tailored application of stem cells according to the pathophysiology of the disease. Stem cell therapy in pediatric diseases has shown promising results and outcomes. However, further studies focusing on its implementation, and optimal treatment time frame are needed. Clinical trials targeting pediatric populations constitute a lower percentage of registered stem cell therapy trials. An increase in preclinical and clinical trials for the treatment of pediatric diseases is required to advance our therapeutic applications.
Adverse effects and complications are of high concern when allogeneic stem cell therapy is used. Although various clinical trials have shown that stem cell therapy is relatively safe, patients are still at risk of serious and potentially life-threatening side effects. Further studies are needed to understand, decrease the incidence, and manage such side effects.
Acknowledgments
Figure 1 was created with https://www.biorender.com/.
Funding: This study was supported by the NIH (No. 5T32GM008792-20).
Footnote
Provenance and Peer Review: This article was commissioned by the Guest Editor (Antonio F. Corno) for the series “The Impact of the Progresses of Knowledge and Technologies in Pediatrics” published in Translational Pediatrics. The article has undergone external peer review.
Reporting Checklist: The authors have completed the Narrative Review reporting checklist. Available at https://tp.amegroups.com/article/view/10.21037/tp-23-92/rc
Peer Review File: Available at https://tp.amegroups.com/article/view/10.21037/tp-23-92/prf
Conflicts of Interest: All authors have completed the ICMJE uniform disclosure form (available at https://tp.amegroups.com/article/view/10.21037/tp-23-92/coif). The series “The Impact of the Progresses of Knowledge and Technologies in Pediatrics” was commissioned by the editorial office without any funding or sponsorship. RES received support for attending meetings and/or travel from University of Texas. CSC reports grants/contracts received from NIH, DOD/MTEC/CDMRP, HopeBio, and Athersys, royalties or licenses from EMIT, Coagulex, and Cellvation, consulting fees from Stream Biomedical, payments or honoraria from CellCare Australia, and Generate Life Sciences, and support for attending meetings and/or travel from CTTACC. CSC has patents planned, issued or pending by University of Texas, participates on advisory board for Duke University, and holds stock or stock options from Cellvation, Inc. The authors have no other conflicts of interests to declare.
Ethical Statement: The authors are accountable for all aspects of the work in ensuring that questions related to the accuracy or integrity of any part of the work are appropriately investigated and resolved.
Open Access Statement: This is an Open Access article distributed in accordance with the Creative Commons Attribution-NonCommercial-NoDerivs 4.0 International License (CC BY-NC-ND 4.0), which permits the non-commercial replication and distribution of the article with the strict proviso that no changes or edits are made and the original work is properly cited (including links to both the formal publication through the relevant DOI and the license). See: https://creativecommons.org/licenses/by-nc-nd/4.0/.
References
- Biehl JK, Russell B. Introduction to stem cell therapy. J Cardiovasc Nurs 2009;24:98-103; quiz 104-5. [Crossref] [PubMed]
- O'Brien T, Barry FP. Stem cell therapy and regenerative medicine. Mayo Clin Proc 2009;84:859-61. [Crossref] [PubMed]
- Kemp KC, Hows J, Donaldson C. Bone marrow-derived mesenchymal stem cells. Leuk Lymphoma 2005;46:1531-44. [Crossref] [PubMed]
- Zhang Y, Huang B. Peripheral blood stem cells: phenotypic diversity and potential clinical applications. Stem Cell Rev Rep 2012;8:917-25. [Crossref] [PubMed]
- Chu DT, Nguyen Thi Phuong T, Tien NLB, et al. Adipose Tissue Stem Cells for Therapy: An Update on the Progress of Isolation, Culture, Storage, and Clinical Application. J Clin Med 2019;8:917. [Crossref] [PubMed]
- Ruhil S, Kumar V, Rathee P. Umbilical cord stem cell: an overview. Curr Pharm Biotechnol 2009;10:327-34. [Crossref] [PubMed]
- Srijaya TC, Ramasamy TS, Kasim NH. Advancing stem cell therapy from bench to bedside: lessons from drug therapies. J Transl Med 2014;12:243. [Crossref] [PubMed]
- Pittenger MF, Discher DE, Péault BM, et al. Mesenchymal stem cell perspective: cell biology to clinical progress. NPJ Regen Med 2019;4:22. [Crossref] [PubMed]
- Thomas S, Jaganathan BG. Signaling network regulating osteogenesis in mesenchymal stem cells. J Cell Commun Signal 2022;16:47-61. [Crossref] [PubMed]
- Harting MT, Srivastava AK, Zhaorigetu S, et al. Inflammation-Stimulated Mesenchymal Stromal Cell-Derived Extracellular Vesicles Attenuate Inflammation. Stem Cells 2018;36:79-90. [Crossref] [PubMed]
- Phinney DG, Pittenger MF. Concise Review: MSC-Derived Exosomes for Cell-Free Therapy. Stem Cells 2017;35:851-8. [Crossref] [PubMed]
- Caplan H, Olson SD, Kumar A, et al. Mesenchymal Stromal Cell Therapeutic Delivery: Translational Challenges to Clinical Application. Front Immunol 2019;10:1645. [Crossref] [PubMed]
- Lalu MM, McIntyre L, Pugliese C, et al. Safety of cell therapy with mesenchymal stromal cells (SafeCell): a systematic review and meta-analysis of clinical trials. PLoS One 2012;7:e47559. [Crossref] [PubMed]
- Fischer UM, Harting MT, Jimenez F, et al. Pulmonary passage is a major obstacle for intravenous stem cell delivery: the pulmonary first-pass effect. Stem Cells Dev 2009;18:683-92. [Crossref] [PubMed]
- Eggenhofer E, Benseler V, Kroemer A, et al. Mesenchymal stem cells are short-lived and do not migrate beyond the lungs after intravenous infusion. Front Immunol 2012;3:297. [Crossref] [PubMed]
- Harting MT, Jimenez F, Xue H, et al. Intravenous mesenchymal stem cell therapy for traumatic brain injury. J Neurosurg 2009;110:1189-97. [Crossref] [PubMed]
- Cuende N, Rico L, Herrera C. Concise review: bone marrow mononuclear cells for the treatment of ischemic syndromes: medicinal product or cell transplantation? Stem Cells Transl Med 2012;1:403-8. [Crossref] [PubMed]
- Kubal C, Sheth K, Nadal-Ginard B, et al. Bone marrow cells have a potent anti-ischemic effect against myocardial cell death in humans. J Thorac Cardiovasc Surg 2006;132:1112-8. [Crossref] [PubMed]
- Koutna I, Peterkova M, Simara P, et al. Proliferation and differentiation potential of CD133+ and CD34+ populations from the bone marrow and mobilized peripheral blood. Ann Hematol 2011;90:127-37. [Crossref] [PubMed]
- Jiang Y, Vaessen B, Lenvik T, et al. Multipotent progenitor cells can be isolated from postnatal murine bone marrow, muscle, and brain. Exp Hematol 2002;30:896-904. [Crossref] [PubMed]
- Roobrouck VD, Clavel C, Jacobs SA, et al. Differentiation potential of human postnatal mesenchymal stem cells, mesoangioblasts, and multipotent adult progenitor cells reflected in their transcriptome and partially influenced by the culture conditions. Stem Cells 2011;29:871-82. [Crossref] [PubMed]
- Highfill SL, Kelly RM, O'Shaughnessy MJ, et al. Multipotent adult progenitor cells can suppress graft-versus-host disease via prostaglandin E2 synthesis and only if localized to sites of allopriming. Blood 2009;114:693-701. [Crossref] [PubMed]
- Walker PA, Bedi SS, Shah SK, et al. Intravenous multipotent adult progenitor cell therapy after traumatic brain injury: modulation of the resident microglia population. J Neuroinflammation 2012;9:228. [Crossref] [PubMed]
- Galli R, Gritti A, Bonfanti L, et al. Neural stem cells: an overview. Circ Res 2003;92:598-608. [Crossref] [PubMed]
- Grochowski C, Radzikowska E, Maciejewski R. Neural stem cell therapy-Brief review. Clin Neurol Neurosurg 2018;173:8-14. [Crossref] [PubMed]
- Einstein O, Karussis D, Grigoriadis N, et al. Intraventricular transplantation of neural precursor cell spheres attenuates acute experimental allergic encephalomyelitis. Mol Cell Neurosci 2003;24:1074-82. [Crossref] [PubMed]
- Pluchino S, Zanotti L, Rossi B, et al. Neurosphere-derived multipotent precursors promote neuroprotection by an immunomodulatory mechanism. Nature 2005;436:266-71. [Crossref] [PubMed]
- Teng YD, Lavik EB, Qu X, et al. Functional recovery following traumatic spinal cord injury mediated by a unique polymer scaffold seeded with neural stem cells. Proc Natl Acad Sci U S A 2002;99:3024-9. [Crossref] [PubMed]
- Ourednik J, Ourednik V, Lynch WP, et al. Neural stem cells display an inherent mechanism for rescuing dysfunctional neurons. Nat Biotechnol 2002;20:1103-10. [Crossref] [PubMed]
- Dalle JH, Duval M, Moghrabi A, et al. Results of an unrelated transplant search strategy using partially HLA-mismatched cord blood as an immediate alternative to HLA-matched bone marrow. Bone Marrow Transplant 2004;33:605-11. [Crossref] [PubMed]
- Barker JN, Krepski TP, DeFor TE, et al. Searching for unrelated donor hematopoietic stem cells: availability and speed of umbilical cord blood versus bone marrow. Biol Blood Marrow Transplant 2002;8:257-60. [Crossref] [PubMed]
- Gluckman E. Milestones in umbilical cord blood transplantation. Blood Rev 2011;25:255-9. [Crossref] [PubMed]
- Styczynski J, Cheung YK, Garvin J, et al. Outcomes of unrelated cord blood transplantation in pediatric recipients. Bone Marrow Transplant 2004;34:129-36. [Crossref] [PubMed]
- Rocha V, Wagner JE Jr, Sobocinski KA, et al. Graft-versus-host disease in children who have received a cord-blood or bone marrow transplant from an HLA-identical sibling. Eurocord and International Bone Marrow Transplant Registry Working Committee on Alternative Donor and Stem Cell Sources. N Engl J Med 2000;342:1846-54. [Crossref] [PubMed]
- Sahai S, Wilkerson M, Xue H, et al. Wharton's Jelly for Augmented Cleft Palate Repair in a Rat Critical-Size Alveolar Bone Defect Model. Tissue Eng Part A 2020;26:591-601. [Crossref] [PubMed]
- Gupta A, El-Amin SF 3rd, Levy HJ, et al. Umbilical cord-derived Wharton's jelly for regenerative medicine applications. J Orthop Surg Res 2020;15:49. [Crossref] [PubMed]
- Smith RR, Barile L, Cho HC, et al. Regenerative potential of cardiosphere-derived cells expanded from percutaneous endomyocardial biopsy specimens. Circulation 2007;115:896-908. [Crossref] [PubMed]
- Ashur C, Frishman WH. Cardiosphere-Derived Cells and Ischemic Heart Failure. Cardiol Rev 2018;26:8-21. [Crossref] [PubMed]
- Davis DR, Zhang Y, Smith RR, et al. Validation of the cardiosphere method to culture cardiac progenitor cells from myocardial tissue. PLoS One 2009;4:e7195. [Crossref] [PubMed]
- Cox CS Jr, Baumgartner JE, Harting MT, et al. Autologous bone marrow mononuclear cell therapy for severe traumatic brain injury in children. Neurosurgery 2011;68:588-600. [Crossref] [PubMed]
- Walker PA, Shah SK, Jimenez F, et al. Intravenous multipotent adult progenitor cell therapy for traumatic brain injury: preserving the blood brain barrier via an interaction with splenocytes. Exp Neurol 2010;225:341-52. [Crossref] [PubMed]
- Bedi SS, Aertker BM, Liao GP, et al. Therapeutic time window of multipotent adult progenitor therapy after traumatic brain injury. J Neuroinflammation 2018;15:84. [Crossref] [PubMed]
- Kota DJ, Prabhakara KS, Toledano-Furman N, et al. Prostaglandin E2 Indicates Therapeutic Efficacy of Mesenchymal Stem Cells in Experimental Traumatic Brain Injury. Stem Cells 2017;35:1416-30. [Crossref] [PubMed]
- Zhang R, Liu Y, Yan K, et al. Anti-inflammatory and immunomodulatory mechanisms of mesenchymal stem cell transplantation in experimental traumatic brain injury. J Neuroinflammation 2013;10:106. [Crossref] [PubMed]
- Chen Y, Li J, Ma B, et al. MSC-derived exosomes promote recovery from traumatic brain injury via microglia/macrophages in rat. Aging (Albany NY) 2020;12:18274-96. [Crossref] [PubMed]
- Wen L, Wang YD, Shen DF, et al. Exosomes derived from bone marrow mesenchymal stem cells inhibit neuroinflammation after traumatic brain injury. Neural Regen Res 2022;17:2717-24. [Crossref] [PubMed]
- Caplan HW, Prabhakara KS, Toledano Furman NE, et al. Combination therapy with Treg and mesenchymal stromal cells enhances potency and attenuation of inflammation after traumatic brain injury compared to monotherapy. Stem Cells 2021;39:358-70. [Crossref] [PubMed]
- Sharma A, Gokulchandran N, Chopra G, et al. Administration of autologous bone marrow-derived mononuclear cells in children with incurable neurological disorders and injury is safe and improves their quality of life. Cell Transplant 2012;21:S79-90. [Crossref] [PubMed]
- Sharma A, Sane H, Gokulchandran N, et al. Intrathecal transplantation of autologous bone marrow mononuclear cells in patients with sub-acute and chronic spinal cord injury: An open-label study. Int J Health Sci (Qassim) 2020;14:24-32. [PubMed]
- Liu G, Zhao Z, Wang H, et al. Therapeutic Efficacy of Human Mesenchymal Stem Cells With Different Delivery Route and Dosages in Rat Models of Spinal Cord Injury. Cell Transplant 2022;31:9636897221139734. [Crossref] [PubMed]
- Yang Y, Pang M, Du C, et al. Repeated subarachnoid administrations of allogeneic human umbilical cord mesenchymal stem cells for spinal cord injury: a phase 1/2 pilot study. Cytotherapy 2021;23:57-64. [Crossref] [PubMed]
- Ruppert KA, Nguyen TT, Prabhakara KS, et al. Human Mesenchymal Stromal Cell-Derived Extracellular Vesicles Modify Microglial Response and Improve Clinical Outcomes in Experimental Spinal Cord Injury. Sci Rep 2018;8:480. [Crossref] [PubMed]
- Liu W, Wang Y, Gong F, et al. Exosomes Derived from Bone Mesenchymal Stem Cells Repair Traumatic Spinal Cord Injury by Suppressing the Activation of A1 Neurotoxic Reactive Astrocytes. J Neurotrauma 2019;36:469-84. [Crossref] [PubMed]
- Lima C, Pratas-Vital J, Escada P, et al. Olfactory mucosa autografts in human spinal cord injury: a pilot clinical study. J Spinal Cord Med 2006;29:191-203; discussion 204-6. [Crossref] [PubMed]
- Lima C, Escada P, Pratas-Vital J, et al. Olfactory mucosal autografts and rehabilitation for chronic traumatic spinal cord injury. Neurorehabil Neural Repair 2010;24:10-22. [Crossref] [PubMed]
- Chen G, Wang Y, Xu Z, et al. Neural stem cell-like cells derived from autologous bone mesenchymal stem cells for the treatment of patients with cerebral palsy. J Transl Med 2013;11:21. [Crossref] [PubMed]
- Cox CS Jr, Juranek J, Kosmach S, et al. Autologous cellular therapy for cerebral palsy: a randomized, crossover trial. Brain Commun 2022;4:fcac131. [Crossref] [PubMed]
- Sun JM, Song AW, Case LE, et al. Effect of Autologous Cord Blood Infusion on Motor Function and Brain Connectivity in Young Children with Cerebral Palsy: A Randomized, Placebo-Controlled Trial. Stem Cells Transl Med 2017;6:2071-8. [Crossref] [PubMed]
- Sun JM, Case LE, Mikati MA, et al. Sibling umbilical cord blood infusion is safe in young children with cerebral palsy. Stem Cells Transl Med 2021;10:1258-65. [Crossref] [PubMed]
- Amanat M, Majmaa A, Zarrabi M, et al. Clinical and imaging outcomes after intrathecal injection of umbilical cord tissue mesenchymal stem cells in cerebral palsy: a randomized double-blind sham-controlled clinical trial. Stem Cell Res Ther 2021;12:439. [Crossref] [PubMed]
- Gu J, Huang L, Zhang C, et al. Therapeutic evidence of umbilical cord-derived mesenchymal stem cell transplantation for cerebral palsy: a randomized, controlled trial. Stem Cell Res Ther 2020;11:43. [Crossref] [PubMed]
- Chang Y, Lin S, Li Y, et al. Umbilical cord blood CD34(+) cells administration improved neurobehavioral status and alleviated brain injury in a mouse model of cerebral palsy. Childs Nerv Syst 2021;37:2197-205. [Crossref] [PubMed]
- Bae SH, Kong TH, Lee HS, et al. Long-lasting paracrine effects of human cord blood cells on damaged neocortex in an animal model of cerebral palsy. Cell Transplant 2012;21:2497-515. [Crossref] [PubMed]
- Infante A, Gener B, Vázquez M, et al. Reiterative infusions of MSCs improve pediatric osteogenesis imperfecta eliciting a pro-osteogenic paracrine response: TERCELOI clinical trial. Clin Transl Med 2021;11:e265. [Crossref] [PubMed]
- Horwitz EM, Prockop DJ, Gordon PL, et al. Clinical responses to bone marrow transplantation in children with severe osteogenesis imperfecta. Blood 2001;97:1227-31. [Crossref] [PubMed]
- Guillot PV, Abass O, Bassett JH, et al. Intrauterine transplantation of human fetal mesenchymal stem cells from first-trimester blood repairs bone and reduces fractures in osteogenesis imperfecta mice. Blood 2008;111:1717-25. [Crossref] [PubMed]
- Jahanbin A, Rashed R, Alamdari DH, et al. Success of Maxillary Alveolar Defect Repair in Rats Using Osteoblast-Differentiated Human Deciduous Dental Pulp Stem Cells. J Oral Maxillofac Surg 2016;74:829.e1-9. [Crossref] [PubMed]
- Nguyen LT, Trieu TTH, Bui HTH, et al. Allogeneic administration of human umbilical cord-derived mesenchymal stem/stromal cells for bronchopulmonary dysplasia: preliminary outcomes in four Vietnamese infants. J Transl Med 2020;18:398. [Crossref] [PubMed]
- Ahn SY, Chang YS, Kim JH, et al. Two-Year Follow-Up Outcomes of Premature Infants Enrolled in the Phase I Trial of Mesenchymal Stem Cells Transplantation for Bronchopulmonary Dysplasia. J Pediatr 2017;185:49-54.e2. [Crossref] [PubMed]
- Pincott ES, Ridout D, Brocklesby M, et al. A randomized study of autologous bone marrow-derived stem cells in pediatric cardiomyopathy. J Heart Lung Transplant 2017;36:837-44. [Crossref] [PubMed]
- Kaushal S, Wehman B, Pietris N, et al. Study design and rationale for ELPIS: A phase I/IIb randomized pilot study of allogeneic human mesenchymal stem cell injection in patients with hypoplastic left heart syndrome. Am Heart J 2017;192:48-56. [Crossref] [PubMed]
- Tarui S, Ishigami S, Ousaka D, et al. Transcoronary infusion of cardiac progenitor cells in hypoplastic left heart syndrome: Three-year follow-up of the Transcoronary Infusion of Cardiac Progenitor Cells in Patients With Single-Ventricle Physiology (TICAP) trial. J Thorac Cardiovasc Surg 2015;150:1198-1207, 1208.e1-2.
- Chen X, Wang C, Yin J, et al. Efficacy of Mesenchymal Stem Cell Therapy for Steroid-Refractory Acute Graft-Versus-Host Disease following Allogeneic Hematopoietic Stem Cell Transplantation: A Systematic Review and Meta-Analysis. PLoS One 2015;10:e0136991. [Crossref] [PubMed]
- Moritani K, Miyawaki R, Tokuda K, et al. Mesenchymal Stem Cell Therapy Overcomes Steroid Resistance in Severe Gastrointestinal Acute Graft-Versus-Host Disease. Case Rep Transplant 2019;2019:7890673. [Crossref] [PubMed]
- Jurado M, De La Mata C, Ruiz-García A, et al. Adipose tissue-derived mesenchymal stromal cells as part of therapy for chronic graft-versus-host disease: A phase I/II study. Cytotherapy 2017;19:927-36. [Crossref] [PubMed]
- Zhang X, Peng Y, Fan Z, et al. Mesenchymal Stem Cells May Ameliorate Nephrotic Syndrome Post-Allogeneic Hematopoietic Stem Cell Transplantation-Case Report. Front Immunol 2017;8:962. [Crossref] [PubMed]
- Shi K, Zhang J, Dong JF, et al. Dissemination of brain inflammation in traumatic brain injury. Cell Mol Immunol 2019;16:523-30. [Crossref] [PubMed]
- Centers for Disease Control and Prevention (CDC). Traumatic Brain Injury (TBI): Incidence and Distribution, 2014. Introduction to Brain Injury-Facts and Stats. 2000. Available online: https://www.cdc.gov/traumaticbraininjury/data/index.html
- Caplan HW, Prabhakara KS, Kumar A, et al. Human cord blood-derived regulatory T-cell therapy modulates the central and peripheral immune response after traumatic brain injury. Stem Cells Transl Med 2020;9:903-16. [Crossref] [PubMed]
- Zhang ZX, Guan LX, Zhang K, et al. A combined procedure to deliver autologous mesenchymal stromal cells to patients with traumatic brain injury. Cytotherapy 2008;10:134-9. [Crossref] [PubMed]
- Noronha NC, Mizukami A, Caliári-Oliveira C, et al. Priming approaches to improve the efficacy of mesenchymal stromal cell-based therapies. Stem Cell Res Ther 2019;10:131. [Crossref] [PubMed]
- Skibber MA, Olson SD, Prabhakara KS, et al. Enhancing Mesenchymal Stromal Cell Potency: Inflammatory Licensing via Mechanotransduction. Front Immunol 2022;13:874698. [Crossref] [PubMed]
- Yamazaki K, Kawabori M, Seki T, et al. Clinical Trials of Stem Cell Treatment for Spinal Cord Injury. Int J Mol Sci 2020;21:3994. [Crossref] [PubMed]
- Cofano F, Boido M, Monticelli M, et al. Mesenchymal Stem Cells for Spinal Cord Injury: Current Options, Limitations, and Future of Cell Therapy. Int J Mol Sci 2019;20:2698. [Crossref] [PubMed]
- Yoon SH, Shim YS, Park YH, et al. Complete spinal cord injury treatment using autologous bone marrow cell transplantation and bone marrow stimulation with granulocyte macrophage-colony stimulating factor: Phase I/II clinical trial. Stem Cells 2007;25:2066-73. [Crossref] [PubMed]
- Syková E, Homola A, Mazanec R, et al. Autologous bone marrow transplantation in patients with subacute and chronic spinal cord injury. Cell Transplant 2006;15:675-87. [Crossref] [PubMed]
- Ra JC, Shin IS, Kim SH, et al. Safety of intravenous infusion of human adipose tissue-derived mesenchymal stem cells in animals and humans. Stem Cells Dev 2011;20:1297-308. [Crossref] [PubMed]
- Romanelli P, Bieler L, Scharler C, et al. Extracellular Vesicles Can Deliver Anti-inflammatory and Anti-scarring Activities of Mesenchymal Stromal Cells After Spinal Cord Injury. Front Neurol 2019;10:1225. [Crossref] [PubMed]
- Saberi H, Moshayedi P, Aghayan HR, et al. Treatment of chronic thoracic spinal cord injury patients with autologous Schwann cell transplantation: an interim report on safety considerations and possible outcomes. Neurosci Lett 2008;443:46-50. [Crossref] [PubMed]
- Levi AD, Anderson KD, Okonkwo DO, et al. Clinical Outcomes from a Multi-Center Study of Human Neural Stem Cell Transplantation in Chronic Cervical Spinal Cord Injury. J Neurotrauma 2019;36:891-902. [Crossref] [PubMed]
- Toyokawa S, Maeda E, Kobayashi Y. Estimation of the number of children with cerebral palsy using nationwide health insurance claims data in Japan. Dev Med Child Neurol 2017;59:317-21. [Crossref] [PubMed]
- Götherström C, Westgren M, Shaw SW, et al. Pre- and postnatal transplantation of fetal mesenchymal stem cells in osteogenesis imperfecta: a two-center experience. Stem Cells Transl Med 2014;3:255-64. [Crossref] [PubMed]
- Tatullo M, Marrelli M, Shakesheff KM, et al. Dental pulp stem cells: function, isolation and applications in regenerative medicine. J Tissue Eng Regen Med 2015;9:1205-16. [Crossref] [PubMed]
- Davidson LM, Berkelhamer SK. Bronchopulmonary Dysplasia: Chronic Lung Disease of Infancy and Long-Term Pulmonary Outcomes. J Clin Med 2017;6:4. [Crossref] [PubMed]
- Ahn SY, Chang YS, Lee MH, et al. Stem cells for bronchopulmonary dysplasia in preterm infants: A randomized controlled phase II trial. Stem Cells Transl Med 2021;10:1129-37. [Crossref] [PubMed]
- Hinton RB, Ware SM. Heart Failure in Pediatric Patients With Congenital Heart Disease. Circ Res 2017;120:978-94. [Crossref] [PubMed]
- Nakano SJ, Miyamoto SD, Price JF, et al. Pediatric Heart Failure: An Evolving Public Health Concern. J Pediatr 2020;218:217-21. [Crossref] [PubMed]
- Koitabashi N, Kass DA. Reverse remodeling in heart failure--mechanisms and therapeutic opportunities. Nat Rev Cardiol 2011;9:147-57. [Crossref] [PubMed]
- Gordon BM, Rodriguez S, Lee M, et al. Decreasing number of deaths of infants with hypoplastic left heart syndrome. J Pediatr 2008;153:354-8. [Crossref] [PubMed]
- Siffel C, Riehle-Colarusso T, Oster ME, et al. Survival of Children With Hypoplastic Left Heart Syndrome. Pediatrics 2015;136:e864-70. [Crossref] [PubMed]
- Altmann K, Printz BF, Solowiejczky DE, et al. Two-dimensional echocardiographic assessment of right ventricular function as a predictor of outcome in hypoplastic left heart syndrome. Am J Cardiol 2000;86:964-8. [Crossref] [PubMed]
- Socié G, Ritz J. Current issues in chronic graft-versus-host disease. Blood 2014;124:374-84. [Crossref] [PubMed]
- Kelly K, Rasko JEJ. Mesenchymal Stromal Cells for the Treatment of Graft Versus Host Disease. Front Immunol 2021;12:761616. [Crossref] [PubMed]
- Jagasia M, Arora M, Flowers ME, et al. Risk factors for acute GVHD and survival after hematopoietic cell transplantation. Blood 2012;119:296-307. [Crossref] [PubMed]
- Le Blanc K, Rasmusson I, Sundberg B, et al. Treatment of severe acute graft-versus-host disease with third party haploidentical mesenchymal stem cells. Lancet 2004;363:1439-41. [Crossref] [PubMed]
- Jerkic M, Szaszi K, Laffey JG, et al. Key Role of Mesenchymal Stromal Cell Interaction with Macrophages in Promoting Repair of Lung Injury. Int J Mol Sci 2023;24:3376. [Crossref] [PubMed]
- Petrus-Reurer S, Romano M, Howlett S, et al. Immunological considerations and challenges for regenerative cellular therapies. Commun Biol 2021;4:798. [Crossref] [PubMed]
- Walker PA, Jimenez F, Gerber MH, et al. Effect of needle diameter and flow rate on rat and human mesenchymal stromal cell characterization and viability. Tissue Eng Part C Methods 2010;16:989-97. [Crossref] [PubMed]