Graft rejection in paediatric congenital heart disease
Introduction
Congenital heart disease (CHD) is the most prevalent global birth defect, with approximately 1.35 million neonates born with abnormalities of the heart and/or blood vessels each year (1). Around a quarter of newborns with CHD need lifesaving surgery before turning 1 year old (2). Due to advancements in cardiac surgical techniques and the advent of novel replacement options for defective or absent cardiovascular structures, prevalence of CHD in adulthood has reached 1 in 150, with around half having had childhood surgery (3). Over 40 CHD subtypes have been defined, ranging from benign to life-threatening defects (4). The right ventricular outflow tract (RVOT) is affected in several CHD pathologies (5), including pulmonary atresia (6), tricuspid atresia (7), double-outlet right ventricle (8), truncus arteriosus (9), pulmonary stenosis (10), univentricular heart (11), and Tetralogy of Fallot (TOF) (12). TOF is characterised by the occurrence of four structural cardiac malformations: overriding aorta, pulmonary stenosis, ventricular septal defect, and right ventricular hypertrophy (12) and is the most common cause of cyanotic CHD, accounting for approximately 3.5% of CHD diagnoses and requiring surgery within the first 6 months of life (12,13). RVOT repair in childhood commonly requires a right ventricle-to-pulmonary artery (RV-PA) replacement, with conduits such as the Contegra bovine jugular vein (BJV) valved conduit (Medtronic) or homografts sourced from a clinical bank (14-16). Whilst replacement with a prosthetic is often lifesaving, no available option is optimal, and repair in infancy does not preclude reintervention at multiple points throughout the patient’s life, with multiple open-heart surgeries in childhood and adulthood commonplace (4). Resultantly, the search for a graft possessing lifelong durability is an ongoing area of paediatric CHD research (17).
A contributor for surgical reintervention is the lack of growth potential of current conduits. Performing corrective surgery at an early age results in the patient inevitably outgrowing the graft and replacement is needed. As such, in situ prosthesis growth is essential if the graft is to last until adulthood (15). However, a more immediate issue facing paediatric CHD patients is graft rejection. Though a significant benefit of the xenograft over its homograft counterpart is accessibility, severe immunological incompatibilities are detrimental obstacles opposing clinical use (18). Hyperacute rejection (HAR) occurs within minutes of implantation in vascularised grafts (19). Caused by the presence of preformed host antibodies, complement activation stimulates production of coagulation factors, resulting in platelet adhesion, aggregation, thrombosis, and ultimately graft degeneration (20). Acute vascular rejection results from a humoral response, namely graft-specific donor antibodies directly targeting the tissue. Acute cellular rejection stems from a targeted immune response with a combination of T-cell and B-cell dependent process (21). Three major epitopes on transplanted animal tissue present the biggest hurdles: galactose-α1,3-galactose (α-Gal), N-glycolylneuraminic acid (Neu5Gc), and SDa. Overall, while limited homograft availability for paediatric repair means xenogenic tissue is the surgical standard, these animal-derived products are not lifelong solutions.
The role of systemic inflammation in rejection following biological prostheses implantation in paediatric CHD patients will be the focus of this review. Calcification of implants, distinguished by tissue mineralisation due to fibrotic remodelling (22), will be discussed as a key mode of graft degeneration. The term “rejection” will be applied in accordance with the Farlex Partner Medical Dictionary, used to describe “the immunologic response to incompatibility in a transplanted organ” (23). This review will first discuss commercially used grafts for paediatric CHD, then explore the diverse roles of innate and adaptive immune response mechanisms in the context of rejection, examining the immune cells involved, downstream signalling cascades, and complement activation. Finally, the review will consider emerging strategies of intervention and look to the future of graft implantation for paediatric patients with CHD.
Utility and function of commercially available cardiac grafts
Prostheses used in surgical interventions are highly susceptible to calcification over time, posing a problem for lasting durability (24,25). In the context of paediatric CHD, where the infant’s inner pulmonary artery diameter is ~5–6 mm, poor patency of the small-diameter vascular grafts used for newborns is problematic, with heightened risk of thrombotic occlusion (26,27). As such, present options available to cardiovascular surgeons are suboptimal.
Cryopreserved pulmonary homografts are the gold standard for RVOT reconstruction, as is required for TOF pathology (5,28,29). While cryopreserved homografts show long-term freedom from replacement in adults, durability in infants is lower, with small size being a risk factor for failure (26,29,30). Despite being deemed the best alternative to the native structure, cryopreserved homografts are still vulnerable to rejection (31-34). Although some degree of allogenic cell removal is achieved by cryopreservation, donor cells are still present and consequently the tissue retains antigenicity and is capable of generating an immune response (35). Notably, cryopreserved allografts promote the induction of anti-human leukocyte antigen (HLA) antibodies within the recipient, with non-valved homograft patches shown to undergo intimal thickening and endothelial activation (36-38), causing increased immune infiltration and a chronic cellular response. Resultantly, the cryopreserved homograft-related immune response has a key role in the rejection of these prostheses in paediatric patients.
Further to considering clinical outcome in paediatric versus adult CHD patients, it is important to further stratify the paediatric population by required conduit size. Evidently, size of cardiac structures rapidly changes in the first years of life, dictating the replacement graft requirements. In a study of 103 infants (5.1±2.8 months) who underwent aortic homograft insertion, patients were divided into groups receiving small (8–11 mm, n=25), medium (12–14 mm, n=45), or large (15–17 mm, n=33) conduits. The average longevity of grafts was 15±4 months for small homografts, 39±7 months for medium, and 50±14 months for large conduits (P<0.001), demonstrating conduit diameter is an independent risk factor for failure (39). A 2021 systematic review of the Ross procedure (n=3,156, median age 9.5 years), found reoperation due to pulmonary homograft failure was heightened in neonates and infants compared to older children, being 4.16–6.58%/year and 0.34–4.76%/year, respectively (40). The precise mechanism behind younger age increasing risk of dysfunction is undetermined, but it is proposed that immune activity and age-related calcium metabolism differences play a role (40). As such, not only must discrepancies between paediatric and adult CHD treatment outcome be considered, but also clinical outcomes within the paediatric population.
Availability of small-diameter homograft conduits is limited, and the scarce availability of paediatric homografts has brought about the need to use a more abundant tissue source (41). Xenografts, commonly porcine or bovine, are an alternative source of biological prostheses for use in CHD intervention (14). To reduce antigenicity of animal tissue, xenografts are treated with glutaraldehyde fixative to crosslink the extracellular matrix (ECM) (42) (Figure 1). The chemical bonds not only decrease antigenicity, but also enhance tissue stability, mechanical resistance, and sterility (42). Nevertheless, by chemically modifying the matrix via fixation with crosslinking agents, graft replacement with neotissue as part of the regenerative healing process is inhibited (43).
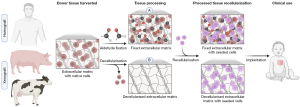
Contegra is a commercially available glutaraldehyde-fixed BJV integrated valved conduit (41,44). Developed in 1999, Contegra is an off-the-shelf product available in a range of sizes and has become the conduit of choice for paediatric RVOT reconstruction where a homograft is not available (16,41). Despite Contegra’s undeniable place as an alternative to the cryopreserved homograft in paediatric CHD, it is not without shortcomings. Clinical data have shown that glutaraldehyde fixative remnants in the tissue can cause strong cytotoxic effects, reducing long-term durability and leading to calcification and endocarditis (25,45). Studies comparing the durability of Contegra against the cryopreserved homograft in paediatric patients have reported contradicting results. While some suggest BJV conduit durability is superior to its homograft counterpart for infant RVOT reconstruction (46-48), others conclude it to be similar (49-51) or inferior to the homograft (52-57). Table 1 summarises clinical data from BJV conduit studies.
Table 1
Study title | Sample size | Patient age | Conduit size | Diagnosis | Study type | Primary outcome | Country |
---|---|---|---|---|---|---|---|
The Contegra bovine valved jugular vein conduit for pediatric RVOT reconstruction: 4 years experience with 108 patients (44) | 108 BJV | Mean age 4.3±5.2 years (range, 2 days–20.1 years); median: 1.8 years | 12–22 mm (median 14 mm) | Congenital heart disease | Observational | No relevant conduit dilatation and no leaflet calcification | Germany |
4-year freedom from reoperation due to conduit inflow or valvular degeneration: 100% | |||||||
4-year freedom from reoperation due to pulmonary artery branch stenoses (n=10): 86% | |||||||
4-year freedom from explantation (n=4): 92% | |||||||
4-year survival: 91.7% | |||||||
Bovine jugular vein conduit: a mid- to long-term institutional review (45) | 315 BJV | 0 to 1 years, n=65; 1–10 years, n=132; 10+ years, n=118 | Small (12/14 mm), n=75; medium (16/18 mm), n=84; large (20/22 mm), n=156 | Congenital heart disease | Observational | 9% early mortality in the 0–1-year-old group, higher (P<0.001) than early mortality in 1–10 year-olds (0%) and 10+ year-olds (1%), P<0.001 | America |
13% 10-year freedom from conduit failure in the 0–1-year-old group (mean time to failure 5.2 years), compared with 53% (mean time to failure 9.9 years) and 69% (mean time to failure 11 years) for the 1–10 and 10+ year-olds, respectively (P<0.001) | |||||||
21 (6.7%) patients developed endocarditis and 11 (3.5%) patients required reoperation, while 10 (3.2%) patients required antibiotic therapy alone | |||||||
Histopathological examination of explanted conduits (n=29, 57% of explants) revealed fibrosis (n=10), diffuse calcification (n=10), focal calcification (n=5), and chronic inflammation (n=5), with no difference in pathologies observed between groups | |||||||
Association of pulmonary conduit type and size with durability in infants and young children (46) | 429 (123 BJV, 275 non-decellularised allografts, 22 decellularised allografts, 9 porcine heterografts) | Mean age 1.7 months (range, 1 day–1.98 years) | BJV: 12–22 mm; allografts: 6–20 mm | Congenital heart disease | Observational | 138 conduit replacements (32%) and 3 explantations (1%) | Canada |
63% conduit durability at a median follow-up of 6.0 years (range, 0.1–11.7 years) | |||||||
Placement of a conduit with smaller z-score was associated with earlier replacement/explantation (P=0.002) | |||||||
BJV provide better durability than an allograft at any indexed size | |||||||
Right ventricular outflow tract reconstruction in infant truncus arteriosus: a 37-year experience (47) | 100 (14 aortic homografts, 41 pulmonary homografts, 36 BJV, 4 valved heterograft conduits, 4 synthetic grafts, 1 direct anastomosis to right ventricle) | Median age 33 days (IQR, 20–71 days) | 12 mm (IQR, 11–12 mm) | Truncus arteriosus | Observational | Actuarial survival at 30 days, 5 years, 10 years, and 15 years was 85%, 72%, 72%, and 68%, respectively | America |
Longer freedom from reoperation was associated with BJV compared with the aortic homograft (hazard ratio, 3.1; 95% CI: 1.3–7.7; P=0.02), with no difference compared with the pulmonary homograft | |||||||
5-year freedom from reoperation was ~65%, ~45%, and ~20% for BJV, pulmonary homograft, and aortic homograft, respectively | |||||||
Larger conduit size was associated with longer freedom from reoperation (hazard ratio, 0.7; 95% CI: 0.6–0.9; P<0.001) | |||||||
Multicenter analysis of early childhood outcomes after repair of truncus arteriosus (48) | 191 (83 pulmonary homografts, 53 aortic homografts, and 55 BJV) | Median age 10 days (IQR, 7–23 days) | Pulmonary homografts: median 10 mm (IQR, 9–11 mm); aortic homografts: median 9 mm (IQR, 9–11 mm); BJV: median 12 mm (IQR, 12–12 mm) | Truncus arteriosus | Observational | 102 patients (53%) required reintervention at a rate of 58% (pulmonary homograft, n=48), 62% (aortic homograft, n=33), and 38% (Contegra, n=21) | America |
Reintervention was not statistically different between homograft types, but likelihood of reintervention was significantly lower for Contegra conduits, independent of conduit diameter | |||||||
Concluded that use of a homograft was an independent risk factor for RV-PA conduit reintervention, as was smaller conduit diameter | |||||||
Pulmonary valve replacement after right ventricular outflow tract reconstruction with homograft vs Contegra®: a case control comparison of mortality and morbidity (49) | 87 (60 BJV, 27 homograft) | 0–18 years | 12–22 mm | Congenital heart disease | Observational | 13.47% (Contegra) vs. 15.36% (homograft) perioperative complication rate, P=0.758 | Belgium |
No difference in blood loss, fluid input, length of ICU stay, length of hospital stay, or postoperative morbidity | |||||||
2.3% total postoperative mortality | |||||||
Contegra versus pulmonary homograft for right ventricular outflow tract reconstruction in newborns (50) | 53 (30 BJV, 23 pulmonary homograft) | BJV: mean age 15±8 days; homograft: mean age 10±7 days | BJV: 12 mm; homograft: 9–14 mm | Congenital heart disease | Observational | 16.6% (BJV) vs. 17.4% (homograft) overall mortality, P=0.98 | Belgium |
No difference in operative morbidity, early reoperation for conduit failure, or survival free from reoperation | |||||||
Can pulmonary conduit dysfunction and failure be reduced in infants and children less than age 2 years at initial implantation? (51) | 241 (160 non-decellularised allografts, 60 BJV, 5 porcine heterografts, and 16 decellularised allografts) | 1.4 months (0–1.95 years) | 12±2 mm | Congenital heart disease | Observational | Constant rate of first conduit intervention, with a prevalence of 58% at 3 years from initial implant | America/Canada |
Common risk-factors for intervention were younger age at initial conduit implant and smaller conduit Z-score | |||||||
Conduit durability and haemodynamic function can be improved by using pulmonary conduits with Z-scores between +1 and +3 | |||||||
Decellularized fresh homografts for pulmonary valve replacement: a decade of clinical experience (52) | 279 (93 decellularised fresh pulmonary homografts, 93 cryopreserved pulmonary homografts, 93 BJV) | Decellularised fresh pulmonary homografts: 15.8±10.21 years; cryopreserved pulmonary homografts: 15.9±10.4 years; BJV: 15.6±9.9 years | Decellularised fresh pulmonary homografts: mean 23.9±4.3 mm; cryopreserved pulmonary homografts: mean 23.3±3.6 mm; BJV: mean 19.9±2.9 mm | Congenital heart disease, with TOF being the most frequent malformation | Observational | 10-year freedom from explantation: 100% for decellularised fresh pulmonary homografts, 84.2% for cryopreserved pulmonary homografts (P=0.01) and 84.3% for BJV (P=0.01) | Germany |
Freedom from infective endocarditis: 100% for decellularised fresh pulmonary homografts, 97.3%±1.9% within matched cryopreserved pulmonary homografts patients (P=0.2), and 94.3%±2.8% for BJV patients (P=0.06) | |||||||
Which type of conduit to choose for right ventricular outflow tract reconstruction in patients below 1 year of age? (53) | 145 (62 homografts, 35 BJV, 48 porcine-valved Dacron conduits) | <1 year | 12.9±1.3 mm | Congenital heart disease | Observational | 5-year freedom from replacement was 69.4%±6.6% (homografts), 59.4%±8.7% (BJV), and 53.8%±7.4% (Dacron), P=0.40 | Germany |
BJV patients experienced moderate conduit stenosis or insufficiency faster than those with a homograft (P=0.01) | |||||||
Age <1 month was a risk factor for conduit replacement in the univariate analysis (P=0.05), but not in the multivariate analysis | |||||||
Mid- to long-term outcomes of bovine jugular vein conduit implantation in Chinese children (54) | 55 BJV | Median age 36 months (range, 1–142 months) | Minimal conduit size calculated according to patient’s weight using standard tables | Congenital heart disease | Observational | BJV failure in 15 patients (29.4%) | China |
Freedom from failure at 1, 3, 5, and 7 years was 98.0%, 85.8%, 76.8%, and 62.1%, respectively | |||||||
9 patients (17.0%) experienced endocarditis | |||||||
Multivariate logistic regression analysis demonstrated endocarditis was a significant risk factor for BJV conduit failure | |||||||
The risk of bacterial endocarditis after percutaneous and surgical biological pulmonary valve implantation (55) | 161 (55 homografts, 106 BJV) | Homografts: mean age 26.2±15.6 years; BJV: mean age 8.1±5.8 years | Homografts: mean diameter 27.4±2.6 mm; BJV: mean diameter 17.4±3.4 mm | Congenital heart disease | Observational | Found bacterial endocarditis in 5 Contegra grafts (4.7%), but no cases in the homograft group | Germany |
High incidence of late infective endocarditis in bovine jugular vein valved conduits (56) | 253 BJV | Median 2 years (15 days–45 years) | Median diameter 16 mm (range, 12–22 mm) |
Congenital heart disease | Observational | A significant increase in endocarditis occurrence was observed in Contegra conduits compared to homografts in the RV-PA position, with an incidence of 10% (n=25) after a median of 7.5 years post-surgery in the former (P<0.001) | America |
77% freedom from endocarditis at 10 years | |||||||
Risk factors for development of endocarditis and reintervention in patients undergoing right ventricle to pulmonary artery valved conduit placement (57) | 792 (410 pulmonary/aortic homografts, 137 porcine heterografts, and 245 BJV) | Pulmonary/aortic homografts: median 3 years (range, 3 days–45 years); porcine heterografts: median 12 years (range, 1–47 years); BJV: median 3 years (range, 15 days–45 years) | Pulmonary/aortic homografts: median 17 mm (range, 8–30 mm); porcine heterografts: median 18 mm (range, 8–30 mm); BJV: median 16 mm (range, 12–22 mm) |
Congenital heart disease | Observational | 23 conduits developed endocarditis (median of 5 years post-surgery) | America |
BJV conduits were associated with a 9-times greater endocarditis risk compared with homografts | |||||||
BJV conduits were associated with a lower risk for replacement (P<0.0002) and reintervention (P<0.0001) | |||||||
Younger age and smaller conduit size were both associated with higher risk of reintervention and replacement | |||||||
Early versus later re-valving in Tetralogy of Fallot with free pulmonary regurgitation - combined cross-sectional and prospective, multi-centre, randomized, parallel-group clinical trial | Recruiting, estimated enrollment 120 | Ages eligible for study: 12 years and older. Repair with TAP before aged 2 years | Adult size conduit (≥18 mm BJV or homograft) | TOF with pulmonary stenosis | Interventional | Primary outcomes: - Mean right ventricular end-diastolic volume indexed to body surface area; - Rate of deceased patients (all-cause mortality) and total number of patients |
Denmark |
New designed ePTFE valved conduits for surgical reconstruction of right-ventricular outflow tract: a multi-centre clinical research | Recruiting, estimated enrollment 200 | Ages eligible for study: child, adult, older adult | N/A | Congenital heart disease | Interventional | Primary outcome: regurgitation index of valved conduit by cardiac magnetic resonance imaging | China |
BJV, bovine jugular vein; RVOT, right ventricular outflow tract; IQR, interquartile range; CI, confidence interval; RV-PA, right ventricle-to-pulmonary artery; ICU, intensive care unit; TOF, Tetralogy of Fallot; TAP, transannular patch; ePTFE, expanded polytetrafluoroethylene; N/A, not available.
As a response to immunogenicity induced by cryopreserved homografts and glutaraldehyde-fixed xenografts, decellularisation of biological grafts was proposed as a solution. The concept of decellularisation is to eliminate donor cells from the tissue while retaining ECM components (58) (Figure 1). Broadly, decellularisation techniques can be categorised as chemical, enzymatic, or physical, with a blend of methods often realising the best outcome (58). Decellularisation has been extensively explored in the literature for both human and animal tissues, including the use of chemical surfactants sodium deoxycholate (59,60), Triton X-100 (59-65), and sodium dodecyl sulphate (59,61-63,66-71), bases such as sodium hydroxide (72), enzymes, for example trypsin (59,60,62,64,65,67,69) and DNase (59,60,62,64,66,69,70) in combination with ethylenediaminetetraacetic acid (EDTA), and/or physical decellularisation methods, for instance supercritical carbon dioxide (73). Though employing a diverse range of reagents, durations, and equipment, the universal aim common to all decellularisation methods is to produce an acellular scaffold with an intact ECM. Decellularisation methodologies have been comprehensively and recently reviewed (18,58).
The first non-glutaraldehyde-fixed conduit was proposed in 1999 by SynerGraft, in which a novel tissue engineering process fabricated acellular tissue then cryopreserved and sterilised prior to implantation (74). In a 10-year comparison of SynerGraft (n=162) and standard cryopreserved (n=124) pulmonary homograft valves for RVOT reconstruction, SynerGraft freedom from dysfunction was 83% at 10 years, significantly higher than the 58% observed in cryopreserved homografts, indicating the SynerGraft decellularisation process increases durability of the conduit (75). However, SynerGraft decellularisation technology was also applied to porcine tissue with catastrophic clinical results (76). SynerGraft decellularised porcine pulmonary valves were implanted in four paediatric patients as the first animal-derived alternative to aldehyde-fixed xenografts in Europe. The severe inflammatory response to the partially decellularised tissue resulted in a 75% mortality rate: one after just 1 week due to valve rupture, and two at 6 and 52 weeks postimplant resulting from critical structural degeneration. The fourth child had the xenograft prophylactically explanted 2 days after implant, and severe inflammation was already present on the explant. Evaluation of grafts explanted at 2 days, 1 week, and 6 weeks showed an early non-specific macrophage- and neutrophil-driven inflammatory response (76). This advanced into a lymphocytic cascade, observed in the 52-week explant. The explants were calcified, but, notably, unused samples of the SynerGraft valves also revealed calcific evidence. No endogenous in vivo recellularisation of the xenografts was observed in explants. As such, though decellularised SynerGraft pulmonary homografts have shown a reduced immunological response in patients (75,77), the same cannot be said for SynerGraft xenografts (76). This disastrous clinical outcome of a non-fixed and only partly decellularised porcine xenograft highlights the danger of immunoincompatibility, warning against implant of incompletely decellularised xenogenic tissue.
This is not to say that decellularisation is inferior to fixation. A direct comparison of decellularised and fixed xenogenic tissue can be seen in the Matrix P Valve (MPV) and Matrix P Plus Valve (MP+V). These commercially available xenografts consist of a decellularised porcine valve in a surrounding equine glutaraldehyde-fixed conduit (78-80). In both paediatric and adult CHD patients, failure of MPV and/or MP+V cardiac grafts has been associated with immune-mediated graft degeneration (78,80), with evidence of fibrosis, severe inflammation, and a foreign-body reaction against leaflets documented (80). A retrospective clinical comparison of the glutaraldehyde-fixed and decellularised component of the MP+V xenografts (n=18) linked tissue-specific antibodies to the incidence of valve insufficiency (78). A further comparison of MP+V components to decellularised allograft valves (n=14) unsurprisingly reported significantly higher IgG levels towards the animal-derived tissue than human. Importantly, the glutaraldehyde-fixed equine conduit gave rise to approximately 2.5-fold higher IgG production than the decellularised porcine valve component. Not only does this confirm a heightened immune response is raised towards xenogenic MP+V compared to decellularised allogenic tissue, but it also points towards decellularisation as a mechanism to reduce the immune rejection response in paediatric CHD.
In the past decade, small intestinal submucosa (SIS) has gained increasing attention and has become one of the bioscaffolds of choice for decellularisation aimed at different surgical applications. SIS has excellent physical properties, including resistance to deformation, ease of handling and shaping, suture retention, good absorbability, and lack of immunogenicity (81). Commercially available decellularised SIS-ECM scaffold materials are predominantly obtained from pig small intestine, with the most widely used in cardiovascular applications being CorMatrix. CorMatrix has received approval by the Food and Drug Administration (FDA) and European authorities for cardiac tissue repair and is being used in various cardiovascular surgery applications, from early correction of paediatric CHD to surgical treatment of acquired heart disease (82). Despite huge initial enthusiasm, CorMatrix has not shown any significant advantages over previous biomaterials, providing variable clinical outcomes. A retrospective study observed that CorMatrix is a safer alternative to conventional patches when employed in atrial and ventricular defect closure; however, when used for arterial vessel reconstruction, is associated with higher reintervention rates as opposed to autologous pericardium (83). Additionally, preclinical studies using the CorMatrix fashioned as a trileaflet valved conduit in a pig model of thoracic aorta replacement showed this model failed to remodel in a structural and anatomical manner, leading to early fibrosis and calcification (84). Similar concerns were raised by studies using this SIS-ECM for valve repair in children (85). To conclude, despite being one of the mostly used materials in paediatric cardiac repair, CorMatrix has not proven to be a definitive solution to CHD corrections.
Mechanisms of rejection and long-term outcomes
While the utility of cardiac xenograft implantation in the context of CHD surgical repair is well documented, the inflammatory responses to xenografts highlight clinical barriers to their use. Bioprosthetic cardiac grafts, whether valves, conduits, or patches, encounter aggressive forms of both humoral and cellular immune system rejection (86-88). Though xenografts are well tolerated in adults and last for >10 years (89), this differs substantially in young adult and paediatric patients, where grafts are expected to last half as long (>5 years) (44,76,90). Though it is worth noting paediatric patients do outgrow their grafts, many encounter premature regurgitation, stenosis, and structural degeneration, unrelated to size (6,90,91). Whilst this degeneration of the grafts will in part be due to the haemodynamic changes faced within the growing patient, deleterious immune responses against xenogenic antigens within the graft are believed to contribute to structural degeneration (62,88,89) and the need for premature reintervention. Thus, the current challenge to CHD treatment is the induction of immune tolerance to the graft.
The implantation of a bioprosthetic valve faces both hyperacute and acute vascular rejection (32,33,92-94). Whilst the graft is fixed in glutaraldehyde to cross-link the xenoantigens present on the surface, rendering them inert, residual xenogenic carbohydrate-based antigens remain exposed, the most investigated of these being α-Gal (95-99). Once suggested to make the grafts essentially immunologically inert, fixed grafts have been shown to incur excessive inflammation and later calcify, with immune infiltrations present (24,100). Although glutaraldehyde may prevent immediate HAR, it does not completely inhibit immune rejection (25,86,101). Additionally, graft inertness prevents integration with the surrounding native tissue, another major impairing factor for long-term graft patency (102). On the other hand, the exposure of carbohydrate xenoantigens might also result from incomplete decellularisation, which represents another immunogenicity source (103).
α-Gal is known to trigger HAR in nonhuman primates (NHP) (98,104,105). Humans have been shown to have circulating α-Gal antibodies in similar levels to that of IgG or IgM (106-108), and this prevalence may explain fast and sustained immune-mediated xenograft failure. While HAR can occur within the first minutes of implantation because of preformed natural antibodies binding epitopes within the graft, cross-linking via glutaraldehyde postpones this, resulting in delayed xenograft rejection (33,98,109,110). Following increased haemodynamic pressures, in part due to size mismatch of the graft due to patient growth, the efficacy of the fixation is further reduced, unmasking more and more xenoantigens. As epitopes become exposed, the almost immediate binding of natural α-Gal antibodies to the antigens expressed on the endothelium of the graft results in damage of the endothelial lining and subsequent accumulation of thrombosis, interstitial haemorrhage, and oedema that disrupts graft function (50,111-114) (Figure 2).
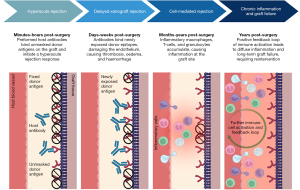
Despite the production of α-Gal knockout (KO) donor animals to negate the presence of the immune response against tissue xenografts, immune-mediated rejection has not been eliminated entirely (98,104,115). A second xenoantigen expressed in native porcine/bovine pericardia is Neu5Gc (116). This ECM-specific glycan has been determined to be present on xenografts and may be responsible for eliciting an inflammatory response (117-119). Explanted xenografts have demonstrated high levels of IgM, IgG and the CD4 complement fragment, indicative of an immunogenic reaction (94,95,120,121). Binding of xenoreactive antibodies activates the complement system through the classical pathway, causing endothelial cell retraction and exposing the underlaying matrix, resulting in the loss of vascular integrity and stimulating the adhesion and activation of platelets, leading to the typical HAR pathology. Classical activation of the complement system begins with antibody-antigen binding. This triggers C1q, activating C1r and C1s, which cleaves C4 and C2 to form C4bC2a, also known as C3 convertase. Following conversion of C3 to C4bC2aC3b (C5 convertase), C5 is cleaved into C5a and C5b, the latter of which works to produce the membrane attack complex, which acts to produce pores within the membrane, leading to cell lysis and rapidly destroying the xenograft (20) (Figure 3).
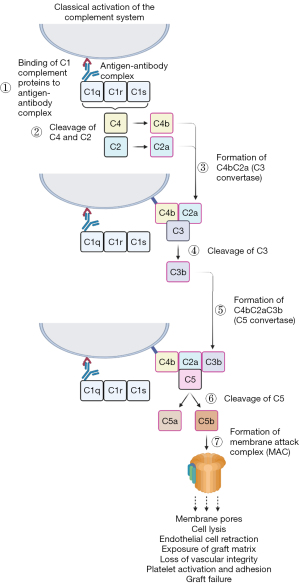
One reason xenografts are susceptible to HAR may be due to the complement regulatory proteins present within the graft being incompatible with the complement system of the recipient, resulting in uncontrolled proliferation of the pathway’s activation. Lack of regulation between porcine and human complement systems contributes significantly to rejection as it allows the activation of the complement to proceed rapidly and unchecked (122). Despite complement activation being a clear detrimental factor contributing to xenograft failure, administering complement inhibitors or upregulating complement regulatory proteins to reduce its activation has been limited due to the enhanced infection risk (32,122). Moreover, adults with implanted xenografts exhibit a significant elevation of α-Gal and Neu5Gc antibody titre following implantation (24). Notably, α-Gal epitopes can be recognised by galectin-3 on monocytes (109), suggesting xenogenic glycans can promote an innate immune response. Further evidence of the xenoantigen-mediated delayed xenograft rejection is seen in comparisons between homografts and xenografts. Homografts exhibit a better performance in adult subjects compared to paediatric (123-125), with the main cause of redo surgery being endocarditis within the graft rather than structural degeneration (55-57,126). However, research is yet to confirm this within a paediatric population. Thrombosis has been well documented in adults implanted with bioprosthetic valves (112,113). Deposition of thrombogenic molecule fibrinogen on graft leaflets has been reported, contributing to recurrent thrombosis formation, triggering chronic inflammation which in turn attracts leukocytes to the xenograft (96,127), promoting further migration, adhesion, and activation of the immune system in direct response to the graft.
Cell-mediated rejection can be seen in xenografts in the months to years following implantation. Accumulation of inflammatory cells, including macrophages, T-cells, and eosinophils, infiltrate the xenograft and contribute to cell-mediated rejection (128,129). In grafts residing in situ for 1+ years, accumulations of eosinophils, predominantly degranulated, were seen within the matrix proximally and distally to its implantation, which would contribute to the inflammatory landscape (85). Moreover, accumulation of infiltrating macrophages and neutrophils promote inflammatory graft degeneration through the production of reactive oxygen species following phagocytosis. This induces oxidative destruction of the graft through loss of collagen and destruction of the glutaraldehyde cross-links, unmasking xenoepitopes and increasing susceptibility for ECM degradation through collagenases (86,130). Immune infiltrates are frequently detected in the areas of ECM deterioration, the breakdown of which is attributed to the proteolytic enzymatic activity of macrophages, themselves attracted to the graft due to the foreign antigens present (85,87,131). Proteolytic macrophage enzymes such as matrix metalloproteases and plasminogen can cleave most ECM proteins, resulting in a positive feedback loop of immune infiltrates (132), breakdown of the implanted graft, further epitope unmasking, and increased inflammation. These proteolytic enzymes are seen in higher levels within implanted grafts compared to native bovine/porcine pericardium, suggesting they are attracted to the graft in situ because of immunological cell-driven rejection. Little research has been conducted on the presence of ECM degrading matrix metalloproteases or cathepsin in paediatric xenografts, and further investigation into this could uncover a mechanism of premature rejection and increased inflammation. Cell-mediated homograft rejection is commonly initiated by a mismatch of allogenic or syngeneic HLA antigens (38,88,133), which mount a targeted T-cell response (93,134), whereas the macrophage response followed by cytotoxic T-cell activation is more crucial in xenografts. Whilst the pro-inflammatory activity of the macrophages, if prolonged, is detrimental for the integrity and integration of the xenograft into the host tissue, this immune cell type can play a pivotal role in graft integration and regeneration. Prevalence of an inflammatory (M1) or regenerative (M2) macrophage phenotype is critical in the response to bioprosthetic grafts. The polarisation towards the M1 or M2 phenotype determines ultimate graft remodelling or regeneration. Cytokines such as interleukin (IL)-4 and IL-10 have been associated with a switch of macrophages from a pro-inflammatory to an anti-inflammatory, pro-healing phenotype. Regulating the macrophage activity and function might provide a strategy for preventing acute inflammation and graft rejection (135).
Calcification often plays a crucial role in longer-term graft failure. Glutaraldehyde fixation is believed to lead to calcification in part due to the chemicals present on the outside of the graft (25,100), namely free aldehyde groups and phospholipids. Moreover, due to the influx of extracellular calcium into the cellular cytosol in direct response to glutaraldehyde treatment, calcified nodules begin to appear on the cell membranes, with concomitant increases in both calcium and inorganic phosphate perpetuating calcification development (42,136). Although cellular mechanisms underlying the association between glutaraldehyde fixation and calcification are not well understood, it has been hypothesised that calcium-containing extracellular fluid influx into devitalised but phosphorus-rich cells might play a role in sxenograft calcification (137). Notably, paediatric patients have a stronger immune system (138) and a higher basal metabolism (139) than the elderly, the two populations which tend to receive xenografts, and that may contribute to the faster graft degeneration seen in the former population. However, pre-treatment of the grafts with ethanol, aluminum-ethanol, or 2-amino oleic acid to inhibit degenerative calcification has not fully eliminated dystrophic calcification within the grafts (140,141), suggesting other mechanisms are at play to result in premature stenosis and/or regurgitation, highlighting the need to further elucidate the role of inflammatory mechanisms in xenograft failure.
Pharmacological treatments and emerging strategies of intervention
As discussed, no existing cardiac replacement option for CHD treatment has demonstrated lifelong durability in paediatric patients, encompassing lasting physiological function free from pathologies such as calcification, thrombosis, endocarditis, and valve regurgitation (41). The search for a widely available, biocompatible, and durable bioprosthetic showing resilience over the patient’s lifetime is ongoing, with multidisciplinary avenues being explored by tissue engineers, clinicians, and basic scientists.
Currently, porcine tissue is deemed the best candidate species for xenotransplantation for multiple reasons; pigs are not endangered, have similar sized and shaped organs to humans, are easy to breed with reasonably large litter sizes, and have suitably fast maturation (21,142). However, phylogenetic distance between pigs and humans results in immunological rejection of xenografts, a phenomena also present, though to a lesser degree, in allografts (21). To this end, an important advantage of pigs for xenotransplantation is the ability to genetically engineer the animals to lack specific genes responsible for rejection (21). Furthering understanding of the immune rejection mechanisms afflicting current prostheses has largely been possible through generating genetically modified (GM) pigs to combat immune incompatibility between mismatched donor species. Genome editing can be achieved by engineered nucleases such as transcription activator-like effector nuclease and zinc finger nuclease (143). The advent of CRISPR/Cas9 systems has seen the rapid production of GM pigs with multiple simultaneous genetic edits (144). As gene editing technologies become more efficient, the production of GM animals will become less arduous and more cost-effective.
The advantage of GM pigs is two-fold: firstly, immunological obstacles limiting xenotransplantation can be understood through observing the host response to donor tissue from a specific gene KO source, and secondly, rejection can be combatted using GM pig tissue for xenotransplantation. The NHP preclinical model is often used as a human proxy in the final step prior to human trials to reveal the mechanisms of rejection of porcine xenografts and evaluate the response to xenotransplants from GM pigs. NHP trials demonstrated α-Gal specific antibodies cause HAR and acute vascular rejection in the host (145). Deletion of the α-Gal epitope has produced a porcine model without this key antigen (99). Later, when hearts from α-Gal KO pigs were transplanted into NHP, acute humoral xenograft rejection still occurred (146). This demonstrated a non-α-Gal-mediated rejection mechanism is also a barrier to xenotransplantation. The SDa blood group is also a critical xenoantigen responsible for immune rejection (147) and was initially identified using α-Gal KO pig complementary DNA expression libraries and screening against NHP serum from animals that rejected the α-Gal KO pig hearts (148). Once discovered, a GM pig lacking the SDa epitope was established through inactivation of the SDa blood group gene, beta-1,4-N-acetyl-galactosaminyltransferase 2 (β4GALNT2) (105). This KO decreases the human non-Gal IgM and IgG response (105). Another option is to genetically modify xenograft donor animals to express human complement regulatory proteins (CD46, CD55, CD59) on their endothelium and this has been an avenue of recent research, with reduced complement deposition seen in both heart and kidney grafts (117,118,149,150). However, as complement regulatory proteins are already produced within the body because of infection to evade host immunity, higher than standard levels might leave patients at higher infection risk, particularly at the graft site. In summary, producing GM pigs is integral to advancing understanding of xenograft rejection, allowing identification and subsequent eradication of xenogenic epitopes. It is important to note that full xenogenic heart transplantation is not currently a viable option due to the need for blank immunosuppression, highlighting the need for alternative strategies such as fixatives.
As discussed, glutaraldehyde-fixation elicits a strong immune response in the patient (78). Increasing efforts have focussed on developing more biocompatible crosslinking agents. It was reported that using polyepoxy compounds to crosslink BJV resulted in a reduction of calcium accumulation and mineralisation when implanted subcutaneously into young rats (151). However, the polyepoxy treatment significantly reduced the strength and stiffness due to hyperhydration of the venous wall (152). Other strategies aimed at improving crosslinking techniques have involved the incorporation of additives to the glutaraldehyde-fixed xenograft, like tripeptide L-glutathione which increased the cytocompatibility (153), or biphosphates immobilised on the jugular vein xenografts, which have shown a calcification inhibitory effect through their ability to block nucleation and prevent the growth of hydroxyapatite crystals (100). The long-term performance of such functionalised grafts is, however, unknown.
Decellularisation of tissue to reduce the immunogenicity of non-autologous biomaterials is proposed as a promising alternative to chemical fixation, and is a constantly evolving field. Yet, complete cell removal in parallel with extensive maintenance of ECM composition is technically challenging. The criteria for effective decellularisation were initially proposed in 2011 (154) and are frequently referenced as the benchmark for success for novel decellularised matrices. These conditions are: (I) <50 nanograms double-stranded DNA per milligram dry weight, (II) DNA fragment length of <200 base pairs present, and (III) no visible nuclear material in haematoxylin and eosin or 4',6-diamidino-2-phenylindole stained tissue sections (154). Though these criteria provide a baseline reference for success, they do not deliver sufficient metrics for complete characterisation. For instance, ECM composition is intrinsically linked to the scaffold’s mechanical properties and is integral to durability (155). Though some degree of ECM disruption is inescapable upon decellularisation, the extent of organisational and compositional alteration regarding macromolecules such as glycosaminoglycans and collagens must be minimised. Additionally, cytotoxicity of decellularisation solution traces, such as sodium dodecyl sulphate, is not considered by the aforementioned measures (155). Legal criteria outlining requirements of an “acellular” scaffold would provide essential safeguarding in the development of novel decellularised tissues for use in paediatric CHD (155); however, precise measures remain undefined (156). New decellularisation approaches endeavour to optimise conditions to maximise maintenance of tissue integrity whilst minimising cellular content. Not only must material origin and composition be considered, but also intended application. The balance between tissue decellularisation and destruction must be found, optimising for enhanced immune tolerance and biocompatibility. Extent of ECM preservation is also donor dependent, meaning standardisation of protocols is potentially unreasonable (157). The ECM not only provides structural integrity, but also contains integral growth factors and signalling molecules for processes including cell recruitment and proliferation (158), further justifying a compromise between cell removal and ECM conservation (157).
In 2021, 0.25% Triton X-100 with 0.25% sodium deoxycholate and 0.02% EDTA, as well as nucleases, was reported to remove native cells from porcine pulmonary valved conduits with minimal ECM damage. Enhancement of scaffold biomimetic properties was achieved by gelatin embedding the decellularised scaffold, followed by 1-ethyl-3-(3-dimethylaminopropyl) carbodiimide (EDC) crosslinking. Gelatin and EDC improved the mechanical properties of the scaffold, including tensile strength, and reduced immunogenicity. The adaptive immune response, namely CD4+ and CD8+ T-cells, is integral to mediating host-graft rejection. Prevalence of CD8+ T-cells was similar between experimental and control valved conduits. CD4+ T-cells were increased in both groups compared to control, though more so in the decellularised than decellularised gelatin-embedded/EDC-crosslinked conduits (159). In line with this, in vivo calcification in an ovine model was less severe in the gelatin-embedded/EDC-crosslinked decellularised conduits than those decellularised without further processing. This study points towards a role of decellularisation followed by gelatin embedding and EDC-crosslinking. Overall, decellularisation of non-self tissue to remove nucleic acids and cellular material such as mitochondria and cell membrane epitopes promises to exceed chemical fixation, which only temporarily masks these foreign immunogens. Once a decellularised scaffold has been obtained, the ECM matrix can be used as an acellular prosthesis for clinical implantation or seeded with cells prior to surgery (Figure 1).
Since mechanical properties are influenced by interpenetrating networks of cells, recellularisation of a fixed or decellularised scaffold is proposed to recapitulate full biocompatibility. In addition to restoring mechanical function, graft recellularisation could enhance immune tolerance, as well as facilitate immediate growth upon implant (14). Recellularisation of a scaffold can be carried out in vitro, prior to implant, or in vivo, relying on the patient’s body to act as a bioreactor for endogenous cell recruitment, followed by infiltration into the scaffold. Recellularisation, whether pre- or post-surgically, has the potential to restore native tissue functions such as growth and repair, facilitating homeostatic graft maintenance. The cell population for seeding needs to be accessible and expandable to clinically useful quantities (27). Regarding the immune response to grafts in paediatric CHD, autologous cells provide a personalised medicine approach to enhance immunocompatibility (14). Mesenchymal stem cells (MSCs) possess multilineage differentiation potential, are highly proliferative, and induce immunotolerance, making them an appealing cell type in cardiac regenerative medicine (160,161). Many MSC sources are suboptimal due to invasive harvesting requirements, such as bone marrow MSCs (162). There are, however, alternative MSC sources that require less invasive isolation. For example, prenatal or early postnatal MSC sources include the thymus (163), chorionic villi (164), and umbilical cord (4). Regarding the latter, autologous MSCs can be isolated non-invasively at the time of birth from Wharton’s jelly (165), cord blood (4,27), or perivascular tissue (166). Once isolated and expanded, these autologous immunoregulatory MSCs can be used to seed scaffolds to produce a recellularised graft ready for implant in early childhood.
In vitro recellularisation of CorMatrix with MSC-derived vascular smooth muscle cells has been explored (27). Following 6 months in piglets, recellularised explants had a homogeneous endothelial layer and multilayer muscular media. In comparison, acellular CorMatrix explants had a sparse incomplete endothelium and thinner muscular media. Importantly, cellular grafts showed growth capacity matching the animal, an efficacy endpoint unmet by the acellular grafts. Though in vitro seeding of the graft holds the capacity to dampen rejection and thus enhance immunocompatibility, recellularised scaffolds need to be freshly prepared for cell viability, precluding long-term storage (167).
An alternative approach to scaffold recellularisation would consist of the functionalisation of the decellularised xenograft with human surface proteins, such as integrins or receptors. These would act as binding sites between the ECM and host cells that would, ultimately, repopulate the graft in vivo (168). The addition of growth factors to the scaffold would further enhance the attraction and binding of endogenous cells, crucial for in vivo recellularisation success, yet this has been little characterised. Similar to cell seeding or ligand immobilisation techniques, some groups have shown that loading decellularised xenografts with immunomodulatory cytokines, such as IL-4 or those within human Wharton’s jelly matrix, could enhance M2 macrophage percentage, resulting in suppressed intimal hyperplasia and enhanced functional regeneration (169,170).
Conclusions
There is currently no perfect solution for the reconstruction of defective paediatric hearts, but xenotransplantation for paediatric CHD treatment holds the potential to overcome the imbalance between small-sized homograft availability and demand. The discovery of new xenoantigens and the advent of novel multi-gene GM pigs as tissue donors, as well as advancements in decellularisation techniques and seeding technology, will enhance biocompatibility and potentially produce a graft unafflicted by rejection issues. Equally, basic research focussed on understanding the molecular mechanisms linking the immune response and calcification to adverse graft remodelling would allow design of therapies aimed at specifically preventing graft rejection.
To address the growing need for replacement cardiac tissue, it will be essential to combine the above approaches to obtain the ideal biological graft, designing the most effective regime for lifelong transplanted tissue functionality. This process begins with the tissue source, be it a homograft, xenograft from a wild-type animal, or xenograft from a GM animal. Tissue processing must be optimised to eliminate donor immunogens, decellularisation solutions and/or glutaraldehyde fixative remnants, as well as retain ECM integrity. In vitro graft functionalisation or autologous cell seeding are other avenues of exploration for enhancing immune compatibility. MSCs are highly relevant immunoprivileged cells for in vitro seeding of grafts intended for use in paediatric CHD treatment and proposed as an ideal cell source for pre-implant cellularisation (171). Surgical techniques are also critical, as well as postoperative care. Use of GM pig-to-NHP xenotransplantation models to assess novel treatment efficacy is also an essential stage in the advent of interventions targeting paediatric CHD. In conclusion, future endeavours will require technological and translational innovation to facilitate tissue engraftment, whilst preventing the risk of acute and chronic rejection and ensuring long-term durability and function.
Acknowledgments
Funding: This work was supported by
Footnote
Provenance and Peer Review: This article was commissioned by the Guest Editors (Antonio F. Corno and Jorge D. Salazar) for the column “Pediatric Heart” published in Translational Pediatrics. The article has undergone external peer review.
Peer Review File: Available at https://tp.amegroups.com/article/view/10.21037/tp-23-80/prf
Conflicts of Interest: All authors have completed the ICMJE uniform disclosure form (available at https://tp.amegroups.com/article/view/10.21037/tp-23-80/coif). The column “Pediatric Heart” was commissioned by the editorial office without any funding or sponsorship. AGH reports grant from the British Heart Foundation PhD studentship (FS/4yPhD/F/20/34125). DI reports grant from the British Heart Foundation (TA/F/21/210028). MC reports grant from British Heart Foundation (AA/18/1/34219, CH/17/1/32804, TA/F/21/210028). FBL reports grant from the Elizabeth Blackwell Institute, University of Bristol, and the Wellcome Trust Institutional Strategic Support Fund (ISSF3, 204813/Z/16/Z). The authors have no other conflicts of interest to declare.
Ethical Statement: The authors are accountable for all aspects of the work in ensuring that questions related to the accuracy or integrity of any part of the work are appropriately investigated and resolved.
Open Access Statement: This is an Open Access article distributed in accordance with the Creative Commons Attribution-NonCommercial-NoDerivs 4.0 International License (CC BY-NC-ND 4.0), which permits the non-commercial replication and distribution of the article with the strict proviso that no changes or edits are made and the original work is properly cited (including links to both the formal publication through the relevant DOI and the license). See: https://creativecommons.org/licenses/by-nc-nd/4.0/.
References
- Alvino VV, Kilcooley M, Thomas AC, et al. In Vitro and In Vivo Preclinical Testing of Pericyte-Engineered Grafts for the Correction of Congenital Heart Defects. J Am Heart Assoc 2020;9:e014214. [Crossref] [PubMed]
- Best C, Strouse R, Hor K, et al. Toward a patient-specific tissue engineered vascular graft. J Tissue Eng 2018;9:2041731418764709. [Crossref] [PubMed]
- Agasthi P, Graziano JN. Catheter Management Pulmonary Valvular Disorders. 2023.
- Swim MM, Albertario A, Iacobazzi D, et al. Amnion-Based Scaffold with Enhanced Strength and Biocompatibility for In Vivo Vascular Repair. Tissue Eng Part A 2019;25:603-19. [Crossref] [PubMed]
- Murala JS, Vela RJ, Geoffrion T, et al. Right ventricular outflow tract obstruction: a quest for ideal management. Asian Cardiovasc Thorac Ann 2018;26:451-60. [Crossref] [PubMed]
- Arunamata A, Goldstein BH. Right ventricular outflow tract anomalies: Neonatal interventions and outcomes. Semin Perinatol 2022;46:151583. [Crossref] [PubMed]
- Feger J, Weerakkody Y. Tricuspid atresia. Radiopaedia.org; 2009.
- Mahle WT, Martinez R, Silverman N, et al. Anatomy, echocardiography, and surgical approach to double outlet right ventricle. Cardiol Young 2008;18:39-51. [Crossref] [PubMed]
- Poaty H, Pelluard F, André G, et al. Truncus arteriosus communis: report of three cases and review of literature. Afr Health Sci 2018;18:147-56. [Crossref] [PubMed]
- Fitzgerald KP, Lim MJ. The pulmonary valve. Cardiol Clin 2011;29:223-7. [Crossref] [PubMed]
- Garcia AM, Beatty JT, Nakano SJ. Heart failure in single right ventricle congenital heart disease: physiological and molecular considerations. Am J Physiol Heart Circ Physiol 2020;318:H947-65. [Crossref] [PubMed]
- Apitz C, Webb GD, Redington AN. Tetralogy of Fallot. Lancet 2009;374:1462-71. [Crossref] [PubMed]
- Rahmath MRK, Boudjemline Y. Tetralogy of Fallot Will be Treated Interventionally Within Two Decades. Pediatr Cardiol 2020;41:539-45. [Crossref] [PubMed]
- Mirani B, Parvin Nejad S, Simmons CA. Recent Progress Toward Clinical Translation of Tissue-Engineered Heart Valves. Can J Cardiol 2021;37:1064-77. [Crossref] [PubMed]
- Durko AP, Yacoub MH, Kluin J. Tissue Engineered Materials in Cardiovascular Surgery: The Surgeon's Perspective. Front Cardiovasc Med 2020;7:55. [Crossref] [PubMed]
- Iyer KS. The Contegra bovine jugular valved conduit: Living up to expectations? Ann Pediatr Cardiol 2012;5:34-5.
- Harris AG, Salih T, Ghorbel MT, et al. Biological Scaffolds for Congenital Heart Disease. Bioengineering (Basel) 2023;10:57. [Crossref] [PubMed]
- Ge F, Lu Y, Li Q, et al. Decellularized Extracellular Matrices for Tissue Engineering and Regeneration. Adv Exp Med Biol 2020;1250:15-31. [Crossref] [PubMed]
- Justiz Vaillant AA, Mohseni M. Chronic Transplantation Rejection. In: StatPearls. Treasure Island (FL): StatPearls Publishing; January 1, 2023.
- Bajic G, Degn SE, Thiel S, et al. Complement activation, regulation, and molecular basis for complement-related diseases. EMBO J 2015;34:2735-57. [Crossref] [PubMed]
- Lu T, Yang B, Wang R, et al. Xenotransplantation: Current Status in Preclinical Research. Front Immunol 2019;10:3060. [Crossref] [PubMed]
- Bartoli-Leonard F, Zimmer J, Aikawa E. Innate and adaptive immunity: the understudied driving force of heart valve disease. Cardiovasc Res 2021;117:2506-24. [Crossref] [PubMed]
- Rejection | definition of rejection by Medical dictionary. Available online: https://www.thefreedictionary.com/
- Senage T, Paul A, Le Tourneau T, et al. The role of antibody responses against glycans in bioprosthetic heart valve calcification and deterioration. Nat Med 2022;28:283-94. [Crossref] [PubMed]
- Grabenwöger M, Sider J, Fitzal F, et al. Impact of glutaraldehyde on calcification of pericardial bioprosthetic heart valve material. Ann Thorac Surg 1996;62:772-7.
- Carrabba M, Madeddu P. Current Strategies for the Manufacture of Small Size Tissue Engineering Vascular Grafts. Front Bioeng Biotechnol 2018;6:41. [Crossref] [PubMed]
- Ghorbel MT, Jia H, Swim MM, et al. Reconstruction of the pulmonary artery by a novel biodegradable conduit engineered with perinatal stem cell-derived vascular smooth muscle cells enables physiological vascular growth in a large animal model of congenital heart disease. Biomaterials 2019;217:119284. [Crossref] [PubMed]
- Van den Eynde J, Sá MPBO, Callahan CP, et al. Right ventricular outflow tract reconstruction with Medtronic Freestyle valve in the Ross procedure: A systematic review with meta-analysis. Artif Organs 2021;45:338-45. [Crossref] [PubMed]
- Boethig D, Goerler H, Westhoff-Bleck M, et al. Evaluation of 188 consecutive homografts implanted in pulmonary position after 20 years. Eur J Cardiothorac Surg 2007;32:133-42. [Crossref] [PubMed]
- Romeo JLR, Mokhles MM, van de Woestijne P, et al. Long-term clinical outcome and echocardiographic function of homografts in the right ventricular outflow tract†. Eur J Cardiothorac Surg 2019;55:518-26. [Crossref] [PubMed]
- Fabian O, Havova M, Gebauer R, et al. Structural Integrity and Cellular Viability of Cryopreserved Allograft Heart Valves in Right Ventricular Outflow Tract Reconstruction: Correlation of Histopathological Changes with Donor Characteristics and Preservation Times. Braz J Cardiovasc Surg 2022;37:639-47. [Crossref] [PubMed]
- González-Gay M, López-Martínez R, Busto-Suárez S, et al. Immunological Aspects Involved in the Degeneration of Cryopreserved Arterial Allografts. Front Surg 2020;7:616654. [Crossref] [PubMed]
- Soquet J, Chambon JP, Goffin Y, et al. Acute rejection of a cryopreserved arterial homograft. Cell Tissue Bank 2015;16:331-3. [Crossref] [PubMed]
- Axelsson I, Malm T. Long-Term Outcome of Homograft Implants Related to Donor and Tissue Characteristics. Ann Thorac Surg 2018;106:165-71. [Crossref] [PubMed]
- Lu T, Huang Y, Liu Y, et al. Effects of cryopreservation on tracheal allograft antigenicity in dogs. J Thorac Dis 2017;9:2038-47. [Crossref] [PubMed]
- Lopes SA, Costa FD, Paula JB, et al. Decellularized heterografts versus cryopreserved homografts: experimental study in sheep model. Rev Bras Cir Cardiovasc 2009;24:15-22. [Crossref] [PubMed]
- Huyan Y, Chang Y, Song J. Application of Homograft Valved Conduit in Cardiac Surgery. Front Cardiovasc Med 2021;8:740871. [Crossref] [PubMed]
- Hawkins JA, Breinholt JP, Lambert LM, et al. Class I and class II anti-HLA antibodies after implantation of cryopreserved allograft material in pediatric patients. J Thorac Cardiovasc Surg 2000;119:324-30. [Crossref] [PubMed]
- Mainwaring RD, Patrick WL, Punn R, et al. Fate of right ventricle to pulmonary artery conduits after complete repair of pulmonary atresia and major aortopulmonary collaterals. Ann Thorac Surg 2015;99:1685-91. [Crossref] [PubMed]
- Moroi MK, Bacha EA, Kalfa DM. The Ross procedure in children: a systematic review. Ann Cardiothorac Surg 2021;10:420-32. [Crossref] [PubMed]
- Qian T, Yuan H, Chen C, et al. Conduits for Right Ventricular Outflow Tract Reconstruction in Infants and Young Children. Front Surg 2021;8:719840. [Crossref] [PubMed]
- Maizato MJ, Higa OZ, Mathor MB, et al. Glutaraldehyde-treated bovine pericardium: effects of lyophilization on cytotoxicity and residual aldehydes. Artif Organs 2003;27:692-4. [Crossref] [PubMed]
- Wong ML, Griffiths LG. Immunogenicity in xenogeneic scaffold generation: antigen removal vs. decellularization. Acta Biomater 2014;10:1806-16. [Crossref] [PubMed]
- Breymann T, Boethig D, Goerg R, et al. The Contegra bovine valved jugular vein conduit for pediatric RVOT reconstruction: 4 years experience with 108 patients. J Card Surg 2004;19:426-31. [Crossref] [PubMed]
- Patel PM, Tan C, Srivastava N, et al. Bovine Jugular Vein Conduit: A Mid- to Long-Term Institutional Review. World J Pediatr Congenit Heart Surg 2018;9:489-95. [Crossref] [PubMed]
- Poynter JA, Eghtesady P, McCrindle BW, et al. Association of pulmonary conduit type and size with durability in infants and young children. Ann Thorac Surg 2013;96:1695-701; discussion 1701-2. [Crossref] [PubMed]
- Herrmann JL, Larson EE, Mastropietro CW, et al. Right Ventricular Outflow Tract Reconstruction in Infant Truncus Arteriosus: A 37-year Experience. Ann Thorac Surg 2020;110:630-7. [Crossref] [PubMed]
- Buckley JR, Amula V, Sassalos P, et al. Multicenter Analysis of Early Childhood Outcomes After Repair of Truncus Arteriosus. Ann Thorac Surg 2019;107:553-9. [Crossref] [PubMed]
- Poinot N, Fils JF, Demanet H, et al. Pulmonary valve replacement after right ventricular outflow tract reconstruction with homograft vs Contegra®: a case control comparison of mortality and morbidity. J Cardiothorac Surg 2018;13:8. [Crossref] [PubMed]
- Falchetti A, Demanet H, Dessy H, et al. Contegra versus pulmonary homograft for right ventricular outflow tract reconstruction in newborns. Cardiol Young 2019;29:505-10. [Crossref] [PubMed]
- Karamlou T, Blackstone EH, Hawkins JA, et al. Can pulmonary conduit dysfunction and failure be reduced in infants and children less than age 2 years at initial implantation? J Thorac Cardiovasc Surg 2006;132:829-38. [Crossref] [PubMed]
- Sarikouch S, Horke A, Tudorache I, et al. Decellularized fresh homografts for pulmonary valve replacement: a decade of clinical experience. Eur J Cardiothorac Surg 2016;50:281-90. [Crossref] [PubMed]
- Vitanova K, Cleuziou J, Hörer J, et al. Which type of conduit to choose for right ventricular outflow tract reconstruction in patients below 1 year of age?†. Eur J Cardiothorac Surg 2014;46:961-6; discussion 966. [Crossref] [PubMed]
- Zhang HF, Chen G, Ye M, et al. Mid- to long-term outcomes of bovine jugular vein conduit implantation in Chinese children. J Thorac Dis 2017;9:1234-9. [Crossref] [PubMed]
- Haas NA, Bach S, Vcasna R, et al. The risk of bacterial endocarditis after percutaneous and surgical biological pulmonary valve implantation. Int J Cardiol 2018;268:55-60. [Crossref] [PubMed]
- Beckerman Z, De León LE, Zea-Vera R, et al. High incidence of late infective endocarditis in bovine jugular vein valved conduits. J Thorac Cardiovasc Surg 2018;156:728-734.e2. [Crossref] [PubMed]
- Mery CM, Guzmán-Pruneda FA, De León LE, et al. Risk factors for development of endocarditis and reintervention in patients undergoing right ventricle to pulmonary artery valved conduit placement. J Thorac Cardiovasc Surg 2016;151:432-9, 441.e1-2.
- García-Gareta E, Abduldaiem Y, Sawadkar P, et al. Decellularised scaffolds: just a framework? Current knowledge and future directions. J Tissue Eng 2020;11:2041731420942903. [Crossref] [PubMed]
- Khorramirouz R, Sabetkish S, Akbarzadeh A, et al. Effect of three decellularisation protocols on the mechanical behaviour and structural properties of sheep aortic valve conduits. Adv Med Sci 2014;59:299-307. [Crossref] [PubMed]
- Yu BT, Li WT, Song BQ, et al. Comparative study of the Triton X-100-sodium deoxycholate method and detergent-enzymatic digestion method for decellularization of porcine aortic valves. Eur Rev Med Pharmacol Sci 2013;17:2179-84.
- Prat-Vidal C, Gálvez-Montón C, Puig-Sanvicens V, et al. Online monitoring of myocardial bioprosthesis for cardiac repair. Int J Cardiol 2014;174:654-61. [Crossref] [PubMed]
- Liao J, Joyce EM, Sacks MS. Effects of decellularization on the mechanical and structural properties of the porcine aortic valve leaflet. Biomaterials 2008;29:1065-74. [Crossref] [PubMed]
- Guhathakurta S, Varghese S, Balasubramanian V, et al. Technique to process xenogenic tissues for cardiovascular implantation - A preliminary report. Current Science 2006;91:1068-73.
- Meyer SR, Chiu B, Churchill TA, et al. Comparison of aortic valve allograft decellularization techniques in the rat. J Biomed Mater Res A 2006;79:254-62. [Crossref] [PubMed]
- Somers P, De Somer F, Cornelissen M, et al. Decellularization of heart valve matrices: search for the ideal balance. Artif Cells Blood Substit Immobil Biotechnol 2012;40:151-62. [Crossref] [PubMed]
- Aldridge A, Desai A, Owston H, et al. Development and characterisation of a large diameter decellularised vascular allograft. Cell Tissue Bank 2018;19:287-300. [Crossref] [PubMed]
- Kumar V, Kumar N, Gangwar AK, et al. Using acellular aortic matrix to repair umbilical hernias of calves. Aust Vet J 2013;91:251-3. [Crossref] [PubMed]
- Tsai TN, Kirton JP, Campagnolo P, et al. Contribution of stem cells to neointimal formation of decellularized vessel grafts in a novel mouse model. Am J Pathol 2012;181:362-73. [Crossref] [PubMed]
- Luo J, Korossis SA, Wilshaw SP, et al. Development and characterization of acellular porcine pulmonary valve scaffolds for tissue engineering. Tissue Eng Part A 2014;20:2963-74. [Crossref] [PubMed]
- Vafaee T, Thomas D, Desai A, et al. Decellularization of human donor aortic and pulmonary valved conduits using low concentration sodium dodecyl sulfate. J Tissue Eng Regen Med 2018;12:e841-53.
- Hennessy RS, Go JL, Hennessy RR, et al. Recellularization of a novel off-the-shelf valve following xenogenic implantation into the right ventricular outflow tract. PLoS One 2017;12:e0181614. [Crossref] [PubMed]
- Campbell EM, Cahill PA, Lally C. Investigation of a small-diameter decellularised artery as a potential scaffold for vascular tissue engineering; biomechanical evaluation and preliminary cell seeding. J Mech Behav Biomed Mater 2012;14:130-42. [Crossref] [PubMed]
- Halfwerk FR, Rouwkema J, Gossen JA, et al. Supercritical carbon dioxide decellularised pericardium: Mechanical and structural characterisation for applications in cardio-thoracic surgery. J Mech Behav Biomed Mater 2018;77:400-7.
- O'Brien MF, Goldstein S, Walsh S, et al. The SynerGraft valve: a new acellular (nonglutaraldehyde-fixed) tissue heart valve for autologous recellularization first experimental studies before clinical implantation. Semin Thorac Cardiovasc Surg 1999;11:194-200.
- Bibevski S, Ruzmetov M, Fortuna RS, et al. Performance of SynerGraft Decellularized Pulmonary Allografts Compared With Standard Cryopreserved Allografts: Results From Multiinstitutional Data. Ann Thorac Surg 2017;103:869-74. [Crossref] [PubMed]
- Simon P, Kasimir MT, Seebacher G, et al. Early failure of the tissue engineered porcine heart valve SYNERGRAFT in pediatric patients. Eur J Cardiothorac Surg 2003;23:1002-6; discussion 1006.
- Brown JW, Ruzmetov M, Eltayeb O, et al. Performance of SynerGraft decellularized pulmonary homograft in patients undergoing a Ross procedure. Ann Thorac Surg 2011;91:416-22; discussion 422-3. [Crossref] [PubMed]
- Böer U, Schridde A, Anssar M, et al. The immune response to crosslinked tissue is reduced in decellularized xenogeneic and absent in decellularized allogeneic heart valves. Int J Artif Organs 2015;38:199-209. [Crossref] [PubMed]
- Konertz W, Angeli E, Tarusinov G, et al. Right ventricular outflow tract reconstruction with decellularized porcine xenografts in patients with congenital heart disease. J Heart Valve Dis 2011;20:341-7.
- Voges I, Bräsen JH, Entenmann A, et al. Adverse results of a decellularized tissue-engineered pulmonary valve in humans assessed with magnetic resonance imaging. Eur J Cardiothorac Surg 2013;44:e272-9. [Crossref] [PubMed]
- Xue A, Sarkeshik A, Boyd WD, et al. Tricuspid Valve Replacement Using CorMatrix® Extracellular Matrix Cylindrical Construct. CTSNeT 2013; [Crossref]
- Mosala Nezhad Z, Poncelet A, de Kerchove L, et al. Small intestinal submucosa extracellular matrix (CorMatrix®) in cardiovascular surgery: a systematic review. Interact Cardiovasc Thorac Surg 2016;22:839-50. [Crossref] [PubMed]
- Weis J, Geiger R, Kilo J, et al. Cormatrix® for vessel reconstruction in paediatric cardiac surgery-a word of caution. Interact Cardiovasc Thorac Surg 2022;34:597-603.
- Mosala Nezhad Z, Poncelet A, de Kerchove L, et al. CorMatrix valved conduit in a porcine model: long-term remodelling and biomechanical characterization. Interact Cardiovasc Thorac Surg 2017;24:90-8. [Crossref] [PubMed]
- Zaidi AH, Nathan M, Emani S, et al. Preliminary experience with porcine intestinal submucosa (CorMatrix) for valve reconstruction in congenital heart disease: histologic evaluation of explanted valves. J Thorac Cardiovasc Surg 2014;148:2216-4, 2225.e1.
- Human P, Bezuidenhout D, Aikawa E, et al. Residual Bioprosthetic Valve Immunogenicity: Forgotten, Not Lost. Front Cardiovasc Med 2021;8:760635. [Crossref] [PubMed]
- Human P, Zilla P. Characterization of the immune response to valve bioprostheses and its role in primary tissue failure. Ann Thorac Surg 2001;71:S385-8. [Crossref] [PubMed]
- Johnston DR, Soltesz EG, Vakil N, et al. Long-term durability of bioprosthetic aortic valves: implications from 12,569 implants. Ann Thorac Surg 2015;99:1239-47. [Crossref] [PubMed]
- Kostyunin AE, Yuzhalin AE, Rezvova MA, et al. Degeneration of Bioprosthetic Heart Valves: Update 2020. J Am Heart Assoc 2020;9:e018506. [Crossref] [PubMed]
- Tiete AR, Sachweh JS, Roemer U, et al. Right ventricular outflow tract reconstruction with the Contegra bovine jugular vein conduit: a word of caution. Ann Thorac Surg 2004;77:2151-6. [Crossref] [PubMed]
- Göber V, Berdat P, Pavlovic M, et al. Adverse mid-term outcome following RVOT reconstruction using the Contegra valved bovine jugular vein. Ann Thorac Surg 2005;79:625-31. [Crossref] [PubMed]
- Qian Z, Hu W, Liu J, et al. Accelerated graft arteriosclerosis in cardiac transplants: complement activation promotes progression of lesions from medium to large arteries. Transplantation 2001;72:900-6. [Crossref] [PubMed]
- Singh AK, Chan JL, DiChiacchio L, et al. Cardiac xenografts show reduced survival in the absence of transgenic human thrombomodulin expression in donor pigs. Xenotransplantation 2019;26:e12465. [Crossref] [PubMed]
- Wu G, Pfeiffer S, Schröder C, et al. Coagulation cascade activation triggers early failure of pig hearts expressing human complement regulatory genes. Xenotransplantation 2007;14:34-47. [Crossref] [PubMed]
- Baumann BC, Forte P, Hawley RJ, et al. Lack of galactose-alpha-1,3-galactose expression on porcine endothelial cells prevents complement-induced lysis but not direct xenogeneic NK cytotoxicity. J Immunol 2004;172:6460-7. [Crossref] [PubMed]
- Baumann BC, Schneider MK, Lilienfeld BG, et al. Endothelial cells derived from pigs lacking Gal alpha(1,3)Gal: no reduction of human leukocyte adhesion and natural killer cell cytotoxicity. Transplantation 2005;79:1067-72. [Crossref] [PubMed]
- Gao B, Long C, Lee W, et al. Anti-Neu5Gc and anti-non-Neu5Gc antibodies in healthy humans. PLoS One 2017;12:e0180768. [Crossref] [PubMed]
- Hisashi Y, Yamada K, Kuwaki K, et al. Rejection of cardiac xenografts transplanted from alpha1,3-galactosyltransferase gene-knockout (GalT-KO) pigs to baboons. Am J Transplant 2008;8:2516-26. [Crossref] [PubMed]
- Phelps CJ, Koike C, Vaught TD, et al. Production of alpha 1,3-galactosyltransferase-deficient pigs. Science 2003;299:411-4. [Crossref] [PubMed]
- Zhuravleva IY, Dokuchaeva AA, Karpova EV, et al. Immobilized Bisphosphonates as Potential Inhibitors of Bioprosthetic Calcification: Effects on Various Xenogeneic Cardiovascular Tissues. Biomedicines 2021;10:65. [Crossref] [PubMed]
- Boudjemline Y, Beyler C, Bonnet D, et al. Surprising outcome similarities between Contegra bovine jugular vein conduit and Shelhigh No-React porcine pulmonary valve conduit: role of immunologic reaction. Eur J Cardiothorac Surg 2003;24:850-1. [Crossref] [PubMed]
- Tan W, Boodagh P, Selvakumar PP, et al. Strategies to counteract adverse remodeling of vascular graft: A 3D view of current graft innovations. Front Bioeng Biotechnol 2022;10:1097334. [Crossref] [PubMed]
- Naso F, Gandaglia A, Iop L, et al. First quantitative assay of alpha-Gal in soft tissues: presence and distribution of the epitope before and after cell removal from xenogeneic heart valves. Acta Biomater 2011;7:1728-34.
- Byrne GW, Stalboerger PG, Davila E, et al. Proteomic identification of non-Gal antibody targets after pig-to-primate cardiac xenotransplantation. Xenotransplantation 2008;15:268-76. [Crossref] [PubMed]
- Estrada JL, Martens G, Li P, et al. Evaluation of human and non-human primate antibody binding to pig cells lacking GGTA1/CMAH/β4GalNT2 genes. Xenotransplantation 2015;22:194-202. [Crossref] [PubMed]
- Galili U. Anti-Gal: an abundant human natural antibody of multiple pathogeneses and clinical benefits. Immunology 2013;140:1-11. [Crossref] [PubMed]
- Cooper DK, Koren E, Oriol R. Oligosaccharides and discordant xenotransplantation. Immunol Rev 1994;141:31-58. [Crossref] [PubMed]
- Helder MRK, Stoyles NJ, Tefft BJ, et al. Xenoantigenicity of porcine decellularized valves. J Cardiothorac Surg 2017;12:56.
- Jin R, Greenwald A, Peterson MD, et al. Human monocytes recognize porcine endothelium via the interaction of galectin 3 and alpha-GAL. J Immunol 2006;177:1289-95. [Crossref] [PubMed]
- Pavlov V, Raedler H, Yuan S, et al. Donor deficiency of decay-accelerating factor accelerates murine T cell-mediated cardiac allograft rejection. J Immunol 2008;181:4580-9. [Crossref] [PubMed]
- Naser JA, Petrescu I, Ionescu F, et al. Gradient changes in bioprosthetic valve thrombosis: duration of anticoagulation and strategies to improve detection. Open Heart 2021;8:e001608. [Crossref] [PubMed]
- Puri R, Auffret V, Rodés-Cabau J. Bioprosthetic Valve Thrombosis. J Am Coll Cardiol 2017;69:2193-211. [Crossref] [PubMed]
- Roudaut R, Serri K, Lafitte S. Thrombosis of prosthetic heart valves: diagnosis and therapeutic considerations. Heart 2007;93:137-42. [Crossref] [PubMed]
- Schoof PH, Koch AD, Hazekamp MG, et al. Bovine jugular vein thrombosis in the Fontan circulation. J Thorac Cardiovasc Surg 2002;124:1038-40. [Crossref] [PubMed]
- Byrne GW, Du Z, Sun Z, et al. Changes in cardiac gene expression after pig-to-primate orthotopic xenotransplantation. Xenotransplantation 2011;18:14-27. [Crossref] [PubMed]
- Tector AJ, Mosser M, Tector M, et al. The Possible Role of Anti-Neu5Gc as an Obstacle in Xenotransplantation. Front Immunol 2020;11:622. [Crossref] [PubMed]
- Foote JB, Jagdale A, Yamamoto T, et al. Histopathology of pig kidney grafts with/without expression of the carbohydrate Neu5Gc in immunosuppressed baboons. Xenotransplantation 2021;28:e12715. [Crossref] [PubMed]
- Iwase H, Jagdale A, Yamamoto T, et al. Evidence suggesting that deletion of expression of N-glycolylneuraminic acid (Neu5Gc) in the organ-source pig is associated with increased antibody-mediated rejection of kidney transplants in baboons. Xenotransplantation 2021;28:e12700. [Crossref] [PubMed]
- Pearce OM, Samraj AN, Läubli H, et al. Reply to Mackenzie: A comparison of Neu5Gc and α-gal xenoantigens. Proc Natl Acad Sci U S A 2015;112:E1405.
- Mathern DR, Horwitz JK, Heeger PS. Absence of recipient C3aR1 signaling limits expansion and differentiation of alloreactive CD8(+) T cell immunity and prolongs murine cardiac allograft survival. Am J Transplant 2019;19:1628-40.
- Miyagawa S, Maeda A, Toyama C, et al. Aspects of the Complement System in New Era of Xenotransplantation. Front Immunol 2022;13:860165. [Crossref] [PubMed]
- Lei T, Chen L, Wang K, et al. Genetic engineering of pigs for xenotransplantation to overcome immune rejection and physiological incompatibilities: The first clinical steps. Front Immunol 2022;13:1031185. [Crossref] [PubMed]
- Boethig D, Thies WR, Hecker H, et al. Mid term course after pediatric right ventricular outflow tract reconstruction: a comparison of homografts, porcine xenografts and Contegras. Eur J Cardiothorac Surg 2005;27:58-66. [Crossref] [PubMed]
- Dittrich S, Alexi-Meskishvili VV, Yankah AC, et al. Comparison of porcine xenografts and homografts for pulmonary valve replacement in children. Ann Thorac Surg 2000;70:717-22. [Crossref] [PubMed]
- Chen R, Duncan JM, Nihill M, et al. Early degeneration of porcine xenograft valves in pediatric patients who have undergone apico-aortic bypass. Tex Heart Inst J 1982;9:41-7.
- Sharma A, Cote AT, Hosking MCK, et al. A Systematic Review of Infective Endocarditis in Patients With Bovine Jugular Vein Valves Compared With Other Valve Types. JACC Cardiovasc Interv 2017;10:1449-58. [Crossref] [PubMed]
- Hogan P, Duplock L, Green M, et al. Human aortic valve allografts elicit a donor-specific immune response. J Thorac Cardiovasc Surg 1996;112:1260-6; discussion 1266-7. [Crossref] [PubMed]
- Goddard MJ, Dunning J. Histopathology of cardiac xenograft rejection in the pig-to-baboon model. J Heart Lung Transplant 2002;21:474-84. [Crossref] [PubMed]
- Davila E, Byrne GW, LaBreche PT, et al. T-cell responses during pig-to-primate xenotransplantation. Xenotransplantation 2006;13:31-40. [Crossref] [PubMed]
- Dalgliesh AJ, Parvizi M, Lopera-Higuita M, et al. Graft-specific immune tolerance is determined by residual antigenicity of xenogeneic extracellular matrix scaffolds. Acta Biomater 2018;79:253-64.
- Liu X, Li N, Gong D, et al. Comparison of detergent-based decellularization protocols for the removal of antigenic cellular components in porcine aortic valve. Xenotransplantation 2018;25:e12380. [Crossref] [PubMed]
- Sakaue T, Nakaoka H, Shikata F, et al. Biochemical and histological evidence of deteriorated bioprosthetic valve leaflets: the accumulation of fibrinogen and plasminogen. Biol Open 2018;7:bio034009. [Crossref] [PubMed]
- Shaddy RE, Hunter DD, Osborn KA, et al. Prospective analysis of HLA immunogenicity of cryopreserved valved allografts used in pediatric heart surgery. Circulation 1996;94:1063-7. [Crossref] [PubMed]
- Singh AK, Chan JL, Seavey CN, et al. CD4+CD25(Hi) FoxP3+ regulatory T cells in long-term cardiac xenotransplantation. Xenotransplantation 2018;25:e12379. [Crossref] [PubMed]
- Browne S, Pandit A. Biomaterial-mediated modification of the local inflammatory environment. Front Bioeng Biotechnol 2015;3:67. [Crossref] [PubMed]
- Kim KM, Herrera GA, Battarbee HD. Role of glutaraldehyde in calcification of porcine aortic valve fibroblasts. Am J Pathol 1999;154:843-52. [Crossref] [PubMed]
- Schoen FJ, Levy RJ. Calcification of tissue heart valve substitutes: progress toward understanding and prevention. Ann Thorac Surg 2005;79:1072-80. [Crossref] [PubMed]
- Simon AK, Hollander GA, McMichael A. Evolution of the immune system in humans from infancy to old age. Proc Biol Sci 2015;282:20143085. [Crossref] [PubMed]
- Vásquez-Alvarez S, Bustamante-Villagomez SK, Vazquez-Marroquin G, et al. Metabolic Age, an Index Based on Basal Metabolic Rate, Can Predict Individuals That are High Risk of Developing Metabolic Syndrome. High Blood Press Cardiovasc Prev 2021;28:263-70. [Crossref] [PubMed]
- Levy RJ, Vyavahare N, Ogle M, et al. Inhibition of cusp and aortic wall calcification in ethanol- and aluminum-treated bioprosthetic heart valves in sheep: background, mechanisms, and synergism. J Heart Valve Dis 2003;12:209-16; discussion 216.
- Ogle MF, Kelly SJ, Bianco RW, et al. Calcification resistance with aluminum-ethanol treated porcine aortic valve bioprostheses in juvenile sheep. Ann Thorac Surg 2003;75:1267-73. [Crossref] [PubMed]
- Cooper DK, Gollackner B, Sachs DH. Will the pig solve the transplantation backlog? Annu Rev Med 2002;53:133-47. [Crossref] [PubMed]
- Wang W, He W, Ruan Y, et al. First pig-to-human heart transplantation. Innovation (Camb) 2022;3:100223. [Crossref] [PubMed]
- Naeimi Kararoudi M, Hejazi SS, Elmas E, et al. Clustered Regularly Interspaced Short Palindromic Repeats/Cas9 Gene Editing Technique in Xenotransplantation. Front Immunol 2018;9:1711. [Crossref] [PubMed]
- Lin SS, Hanaway MJ, Gonzalez-Stawinski GV, et al. The role of anti-Galalpha1-3Gal antibodies in acute vascular rejection and accommodation of xenografts. Transplantation 2000;70:1667-74. [Crossref] [PubMed]
- Kuwaki K, Tseng YL, Dor FJ, et al. Heart transplantation in baboons using alpha1,3-galactosyltransferase gene-knockout pigs as donors: initial experience. Nat Med 2005;11:29-31. [Crossref] [PubMed]
- Byrne G, Ahmad-Villiers S, Du Z, et al. B4GALNT2 and xenotransplantation: A newly appreciated xenogeneic antigen. Xenotransplantation 2018;25:e12394. [Crossref] [PubMed]
- Byrne GW, Stalboerger PG, Du Z, et al. Identification of new carbohydrate and membrane protein antigens in cardiac xenotransplantation. Transplantation 2011;91:287-92.
- Lu TY, Xu XL, Du XG, et al. Advances in Innate Immunity to Overcome Immune Rejection during Xenotransplantation. Cells 2022;11:3865. [Crossref] [PubMed]
- Montgomery RA, Stern JM, Lonze BE, et al. Results of Two Cases of Pig-to-Human Kidney Xenotransplantation. N Engl J Med 2022;386:1889-98. [Crossref] [PubMed]
- Zhuravleva IY, Nichay NR, Kulyabin YY, et al. In search of the best xenogeneic material for a paediatric conduit: an experimental study. Interact Cardiovasc Thorac Surg 2018;26:738-44. [Crossref] [PubMed]
- Zhuravleva IY, Karpova EV, Dokuchaeva AA, et al. Bovine jugular vein conduit: What affects its elastomechanical properties and thermostability? J Biomed Mater Res A 2022;110:394-408. [Crossref] [PubMed]
- Jiang Z, Wu Z, Deng D, et al. Improved Cytocompatibility and Reduced Calcification of Glutaraldehyde-Crosslinked Bovine Pericardium by Modification With Glutathione. Front Bioeng Biotechnol 2022;10:844010. [Crossref] [PubMed]
- Crapo PM, Gilbert TW, Badylak SF. An overview of tissue and whole organ decellularization processes. Biomaterials 2011;32:3233-43. [Crossref] [PubMed]
- Kawecki M, Łabuś W, Klama-Baryla A, et al. A review of decellurization methods caused by an urgent need for quality control of cell-free extracellular matrix' scaffolds and their role in regenerative medicine. J Biomed Mater Res B Appl Biomater 2018;106:909-23.
- Scarritt ME, Pashos NC, Bunnell BA. A review of cellularization strategies for tissue engineering of whole organs. Front Bioeng Biotechnol 2015;3:43. [Crossref] [PubMed]
- Tenreiro MF, Almeida HV, Calmeiro T, et al. Interindividual heterogeneity affects the outcome of human cardiac tissue decellularization. Sci Rep 2021;11:20834. [Crossref] [PubMed]
- Moore MJ, Tan RP, Yang N, et al. Bioengineering artificial blood vessels from natural materials. Trends Biotechnol 2022;40:693-707. [Crossref] [PubMed]
- Wan J, Zhong X, Xu Z, et al. A decellularized porcine pulmonary valved conduit embedded with gelatin. Artif Organs 2021;45:1068-82. [Crossref] [PubMed]
- Ong CS, Yesantharao P, Huang CY, et al. 3D bioprinting using stem cells. Pediatr Res 2018;83:223-31. [Crossref] [PubMed]
- Brown MA, Rajamarthandan S, Francis B, et al. Update on stem cell technologies in congenital heart disease. J Card Surg 2020;35:174-9. [Crossref] [PubMed]
- Zazzeroni L, Lanzoni G, Pasquinelli G, et al. Considerations on the harvesting site and donor derivation for mesenchymal stem cells-based strategies for diabetes. CellR4 Repair Replace Regen Reprogram 2017;5:e2435.
- Iacobazzi D, Swim MM, Albertario A, et al. Thymus-Derived Mesenchymal Stem Cells for Tissue Engineering Clinical-Grade Cardiovascular Grafts. Tissue Eng Part A 2018;24:794-808. [Crossref] [PubMed]
- Schmidt D, Mol A, Breymann C, et al. Living autologous heart valves engineered from human prenatally harvested progenitors. Circulation 2006;114:I125-31. [Crossref] [PubMed]
- Iacobazzi D, Rapetto F, Albertario A, et al. Wharton's Jelly-Mesenchymal Stem Cell-Engineered Conduit for Pediatric Translation in Heart Defect. Tissue Eng Part A 2021;27:201-13. [Crossref] [PubMed]
- Latifi N, Lecce M, Simmons CA. Porcine Umbilical Cord Perivascular Cells for Preclinical Testing of Tissue-Engineered Heart Valves. Tissue Eng Part C Methods 2021;27:35-46. [Crossref] [PubMed]
- Huang K, Ozpinar EW, Su T, et al. An off-the-shelf artificial cardiac patch improves cardiac repair after myocardial infarction in rats and pigs. Sci Transl Med 2020;12:eaat9683. [Crossref] [PubMed]
- Massaro MS, Pálek R, Rosendorf J, et al. Decellularized xenogeneic scaffolds in transplantation and tissue engineering: Immunogenicity versus positive cell stimulation. Mater Sci Eng C Mater Biol Appl 2021;127:112203. [Crossref] [PubMed]
- Tan RP, Chan AHP, Wei S, et al. Bioactive Materials Facilitating Targeted Local Modulation of Inflammation. JACC Basic Transl Sci 2019;4:56-71. [Crossref] [PubMed]
- Gupta P, Chaudhuri GR, Janani G, et al. Functionalized Silk Vascular Grafts with Decellularized Human Wharton's Jelly Improves Remodeling via Immunomodulation in Rabbit Jugular Vein. Adv Healthc Mater 2021;10:e2100750. [Crossref] [PubMed]
- Uccelli A, Moretta L, Pistoia V. Mesenchymal stem cells in health and disease. Nat Rev Immunol 2008;8:726-36. [Crossref] [PubMed]