A narrative review on treatment strategies for neonatal hypoxic ischemic encephalopathy
Introduction
Hypoxic-ischemic encephalopathy (HIE) is a syndrome of brain dysfunction resulting from inadequate oxygenation and perfusion around the time of birth (1). In term infants, HIE has a prevalence of about 1 in every 1,000 births in resource-rich countries (2,3) and 5–40 in every 1,000 births in resource-limited countries (4-7). Overall, HIE is among the leading causes of neonatal death (8,9) and is a major contributor to world-wide disability. Up to 60% of infants with severe HIE die or develop profound disability (2,10-13). In infants born at term or post-term, abnormal neurological function typically becomes evident within the first few hours after birth. Symptoms can range from a reduced level of consciousness, seizures, change in muscle tone and reflexes, to difficulties initiating and maintaining respiration (1,14,15). While HIE also occurs in preterm infants, due to differences in pathophysiology (16-20), this narrative review solely focuses on HIE in term infants born after 36 weeks.
Sarnat & Sarnat introduced the first systematic approach to define distinguishing features of HIE into stages of severity over the first hours to weeks to determine prognosis (14). This was later adapted to the “Modified Sarnat Score” to identify HIE severity within the first 6 hours of injury (21). The initial assessment of a potential HIE case involves a comprehensive examination, combining both clinical and biochemical tests. Clinicians evaluate the infant’s history, Apgar scores, cord pH or postnatal blood gas pH, and whether there was a need for respiratory support lasting more than 10 minutes. This information, coupled with a neurological exam, assists in classifying the severity of HIE into mild, moderate, or severe encephalopathy (2,22,23).
Conditions that lead to HIE and can affect HIE severity are referred to as “intrapartum hypoxic events”, or “intrapartum-related complications”. Events that significantly increase the risk of HIE are placental abruption, placental insufficiency or infarction, maternal anemia, perinatal oligohydramnios, cord prolapse, or nuchal cord (2). Similar terminologies used to describe HIE include “perinatal asphyxia”, “birth asphyxia” or “post-asphyxia encephalopathy”. In cases when there is no evidence of a hypoxic and/or ischemic event, the nonspecific terminology of “neonatal encephalopathy” is used (24,25). In fact, a minority of neonatal encephalopathy cases in term infants stem from non-hypoxic/ischemic causes, which can include intracranial infections, intracranial hemorrhage, hypoglycemia, kernicterus, metabolic disorders, inborn error of metabolism and malignant epilepsy syndromes (25,26). Given that these conditions require different interventions than HIE, accurate diagnosis becomes essential (27-29).
Therapeutic hypothermia (TH) is the only Food and Drug Administration approved treatment for HIE. Following numerous preclinical studies (30-32), TH became widely used after the publication of three landmark randomized controlled trials between 2005 and 2009: the National Institute of Child Health and Development (NICHD) (21), the CoolCap (33) and the TOBY (34) trials. TH involves cooling the infant within 6 h of the intrapartum hypoxic event to 33–34 ℃ for 72 hours. This is followed by a gradual re-warming by 0.5 ℃/h until the core temperature is maintained at 36.5–37.0 ℃ (Table 1) (35,36). TH has emerged as the standard of care for moderate to severe HIE (2,35,37), and though mild cases were not included in these landmark trials, some medical centers in America and Europe also utilize TH in mild HIE cases (38). However, outcomes of TH for mild HIE have been mixed (39-41), and further research is needed to ascertain the effectiveness and safety of TH in the treatment of mild HIE, which is underway with the TIME study (NCT04176471) (42).
Table 1
General criteria | Inclusion criteria |
---|---|
Gestational age | ≥36 weeks |
Birth weight | ≥1,800 g |
Age at initiation | ≤6 hours |
Apgar score | ≤5 at 10 minutes |
Acidosis | Cord pH ≤7.0 or base deficit ≥16 mmol/L |
Evidence of moderate to severe encephalopathy | Yes (modified Sarnat staging) or Seizure |
Depth | Target core temperature of 33.5 ℃ (range: 32–34 ℃) for whole-body cooling; 34–35 ℃ for selective head cooling |
Duration | 72 hours of cooling, followed by a slow rewarming process (0.5 ℃/hour) |
A multi-country open-label, randomized controlled trial (HELIX) revealed that TH in low resource countries was not effective and could even be detrimental (43). This disparity may possibly be due to difficulty in diagnosis and stratification of HIE, or by an inability to identify variability in the mechanism and timing of the hypoxic event (i.e., intermittent hypoxia during labor vs. a single, acute hypoxic event) (43-46). All in all, TH provides incomplete neuroprotection, has a narrow time window for treatment, can cause side effects, including cardiac, liver, kidney and bone marrow dysfunction (18,29). TH is also not readily available in all clinical settings and may have reduced efficacy in low-income settings (45,46). Understanding the cellular and molecular mechanisms behind acute and long-term injury after HIE will facilitate the development of more effective implementation of TH for HIE, and for new therapies. We present this article in accordance with the Narrative Review reporting checklist (available at https://tp.amegroups.com/article/view/10.21037/tp-23-253/rc).
Methods
We comprehensively evaluated clinical studies reporting outcomes after HIE prior to TH using PubMed and Google Scholar databases and “neonatal hypoxia”, “neonatal hypoxic ischemia”, “birth asphyxia”, “hypoxic ischemic encephalopathy”, “term”, and “therapeutic hypothermia” as key words. Studies that reported long-term outcomes after HIE were included in this review. To discuss the mechanisms of injury after HIE, we include papers that used large and small animal models that represent hypoxia-ischemia in a term equivalent brain. Next, we highlight the landmark studies that led to the adoption of TH as the standard of care, and the ongoing work to optimize its use. Finally, we explore emerging therapeutics entering phase II clinical trials and their mechanisms of action. The methods of research used in this narrative review are detailed in Table 2.
Table 2
Items | Specification |
---|---|
Date of search | September 1st, 2022 to August 2nd, 2023 |
Databases and other sources searched | PubMed, Google Scholar |
Search terms used | “neonatal hypoxia”, “neonatal hypoxic ischemia”, “birth asphyxia”, “hypoxic ischemic encephalopathy”, “term”, and “therapeutic hypothermia” |
Timeframe | 1948–2023 |
Inclusion and exclusion criteria | English language studies were included |
Selection process | Independently selected by authors |
Discussion
Phases of injury in hypoxic ischemic encephalopathy
The developing brain undergoes dramatic changes during the early post-natal stages, characterized by the proliferation of astrocytes, synaptogenesis and pruning, arborization, and extensive myelination that persists into early adulthood (47). Unfortunately, HIE disrupts these carefully orchestrated processes resulting in persistent abnormal neurological development and function for survivors. The brain regions most susceptible to injury are the areas of greatest metabolic demand and of highest oxygen and glucose deprivation (48). Human studies utilizing 18F-fluorodeoxyglucose positron emission tomography have provided insights into brain metabolic demand, revealing that term infants have the highest metabolic activity in the thalamus, brain stem, and cerebellar vermis (49-52). The high metabolic demand of these regions also renders them vulnerable to excitotoxicity, free radical production, mitochondrial damage (31,48), and white matter injury (53). This aligns with findings from Magnetic Resonance Imagining (MRI) during acute injury (54), which demonstrate a characteristic pattern of injury in the subcortical regions of the hippocampus, thalamus, and basal ganglia (55). Later, during the subacute phase (1–30 days), MRI injury patterns involve the intraarterial watershed regions in the subcortical white matter (55) as a result of preferential shunting of blood to vital brain structures such as the thalamus, basal ganglia, hippocampi, brain stem, and cerebellar vermis (56). MRI has important prognostic ability for outcomes at 6–7 years of age, with a normal MRI reassuring of normal development (57). The worst prognosis is predominantly seen in infants who have injuries in the basal ganglia, thalamus, the anterior and posterior limbs of the internal capsule in combination with additional cerebral lesions, or in infants with hemispheric devastation (57). Interestingly, even mild cases of HIE have been found to have smaller volumes of the left and right basal ganglia compared to age and sex matched controls (55), which are associated with neurodevelopmental deficits at 18 months (58) to 7 years (59). While these patterns of injury provide important clinical guidance, they are the consequence of targetable molecular and cellar responses to HI injury, which follows a sequence of acute, latent, secondary, and tertiary phases.
The acute phase (0–6 hours post-injury) is dominated by the direct response to oxygen and glucose deprivation (Figure 1). Cells initially compensate by undergoing adaptive cellular responses, including anaerobic glycolysis, measured by accumulation of lactate and pyruvate ions and an early formation of free radicals such as super oxide, non-protein bound iron (60) and nitric oxide (NO) (54). Eventually, the decrease of adenosine triphosphate (ATP) disrupts the release and uptake of excitatory amino acids (glutamate) leading to excitotoxicity in both neurons and glia. Excitotoxicity results from tonic depolarization that occurs via N-methyl-D-aspartate (NMDA) and indirectly with α-amino-3-hydroxy-5-methyl-4-isoxazolepropionic acid (AMPA) receptors, leading to intracellular calcium ion accumulation. This results in necrosis of cells and inflammatory release of products that trigger “regulated cell death” pathways. The cellular mechanisms of death after hypoxic ischemic injury in neonates is reviewed by Thornton et al. (61). The damage-associated molecular patterns (DAMPs) released from the acute phase initiate widespread cascades of programmed cell death and inflammation. This secondary damage is greater than the initial injury, is marked by the emergence of seizure activity, and it is delayed hours after the onset of ischemia (62). This delay before the second phase of injury is called latent phase (see below) and offers an ideal time for neuroprotective treatment (Figure 1).
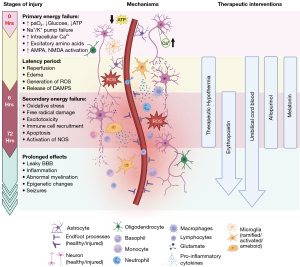
The transition into the “latent phase” occurs when there is a restoration of blood flow and oxygenation. Accumulated lactate and pyruvate ions increase reactive oxygen species (ROS+) leading to damage and permeability changes in the blood brain barrier (BBB), resulting in edema and leakage (63-65). These effects culminate into the secondary energy failure that occurs 6–15 hours after injury, and lasts 24–72 hours (Figure 1). This phase is characterized by progressive neuronal injury, excitotoxicity, and inflammation. As with the first wave of energy failure, this second wave is marked by an increase in intracellular calcium. NO synthase (NOS) releases NO in a calcium dependent manner by glial cells, neuronal (nNOS) and endothelial cells (eNOS), and NOS acts in a calcium independent manner in its immunologic (iNOS) isoforms (66,67). iNOS will persistently produce NO after the initial injury (68). NO perpetuates free radical generation through cyclooxygenase activity and can combine with superoxide to form peroxynitrite, an activator of lipid peroxidation which results in membrane leakage. Additionally, there is an increase in free radical production (superoxide, hydrogen peroxide, iron), which accumulate until it overwhelms the endogenous antioxidant systems resulting in lipid membrane fragmentation by peroxidation (63,69). After the second wave has passed, the extent of injury appears to stabilize but does not resolve.
The tertiary phase (72 hours to several weeks post-injury) is marked by repair and regeneration of damaged neurons and glia (Figure 1). While the ongoing injury during this stage is not as marked as the initial injury, there are still ongoing inflammatory processes and persistent changes in cellular function. There is persistent BBB damage, allowing the passage of otherwise tightly regulated substances, such as IgG proteins, albumin, and inflammatory cells into the brain. These contribute to an upregulation of inflammatory cytokines and to epigenetic changes in brain gene expression, which further contribute to long term neurological dysfunction (70).
Clinical outcomes in the era prior to therapeutic hypothermia
Prior to the widespread adoption of TH in tertiary medical centers, a few studies, conducted between the mid-1980s and 2010s, evaluated the short- and long-term outcomes of neonates with HIE, classified into mild, moderate, or severe subtypes. During this period, resuscitation was the only available intervention. These studies brought to light the acute consequences of HIE, including seizures and death, and highlighted the long-term neurological sequelae, regardless of HIE subtype. Early reports on the outcomes of infants with HIE did not have specific inclusion criteria, making it difficult to determine the exact extent of disability. However, mortality for severe HIE ranged from 15% to 82%, while for moderate HIE it was reported to be 5% (71-73). Reported motor disability rates ranged from 6% to 21% (71,74) for infants with moderate HIE and from 42% to 100% (71,74) for those with severe encephalopathy (Table 3). Notably, even children with HIE who did not exhibit any obvious neurological deficits and even with mild HIE were found to have a range of long-term cognitive (10,11,71,72,74,75), and neurodevelopmental impairments (10-12,74), such as Attention-Deficit Hyperactivity Disorder and Autism Spectrum Disorder (Table 3). These studies highlighting the profound physical and cognitive sequelae of HIE underscored the urgent need for the development and implementation of therapeutic interventions. In the 1940s–50s, researchers began to investigate the potential of hypothermia in treating infants with severe hypoxic injury (76,77). Initially, the practical application of cooling resulted in complications and poor outcomes for patients (78). However, the accumulation of numerous pre-clinical studies allowed for the optimization of the cooling protocol, resulting in a safe and effective translation of this therapeutic approach to human populations (79).
Table 3
Reference | Age (weeks) | Timepoint (years) | Study size | % Mortality | % Morbidity: motor | Morbidity: cognitive | Morbidity: neurodevelopmental impairment | |||||||||||||||||
---|---|---|---|---|---|---|---|---|---|---|---|---|---|---|---|---|---|---|---|---|---|---|---|---|
Control | HIE | HIE | HIE | Control [SD] | HIE | Control | HIE | |||||||||||||||||
Mild | Moderate | Severe | Mild | Moderate | Severe | Mild | Moderate | Severe | Mild [SD] | Moderate [SD] | Severe [SD] | Mild | Moderate | Severe | ||||||||||
Robertson & Finer, 1985 | ≥37 | 3.5 | NA | 66 | 94 | 7 | 0% | 5% | 75% | 0 | 21% | 100% | NA | SB102 [14] | SB92 [23] | SB37 [27] | NA | NA | NA | NA | ||||
Shankaran et al., 1991 | 38–42 | 5 | NA | NA | NA | 28 | NA | NA | 15% | NA | NA | 42% | NA | NA | NA | GCI50% | NA | NA | NA | NA | ||||
Robertson et al., 2003 | ≥37 | 8 | 155 | 56 | 90 | 28 | 0 | 5% | 82% | NA | 15% | a15% | a16% | a35% | a100% | NA | NA | NA | NA | |||||
Marlow et al., 2005 | ≥35 | 7 | 49 | NA | 34 | 31 | NA | NA | NA | NA | 6% | 42% | b114 [14] | NA | b112 [11] | b103 [13] | c9% | NA | c16% | c50% | ||||
Badawi et al., 2006 | ≥37 | 5 | 564 | NA | 276 | NA | 13% | NA | NA | NA | NA | NA | NA | NA | ASD0.8% | NA | ASD5% | |||||||
Lindström et al., 2006 | dterm | 15–19 | 15 | NA | d28 | NA | NA | NA | NA | NA | NA | NA | WISC0% | NA | WISC,d64% | NA | e0% | NA | e36% | NA | ||||
van Handel et al., 2010 | 37–42 | 9–10 | 53 | 34 | 47 | NA | NA | NA | NA | NA | NA | NA | WISC109 [12] | WISC98 [12] | WISC87 [22] | NA | CBCL8% | CBCL18% | CBCL21% | NA |
a, the highest % of affected patients/HIE subtype for reading, spelling and arithmetic delay is reported. Note that at 8-year follow-up the study size was 56 for mild, 84 for moderate and 5 for severe HIE; b, note that only patients with HIE without motor disabilities were included (32 with moderate and 18 with severe encephalopathy); c, behaviour was assessed using the strengths and difficulties questionnaire, reported are the parent reports (note that 6 were missing), % refers to the percentage of abnormal scores per group; d, for this study age in weeks was not specified, only patients without motor disabilities were included, the WISC was available for 11/28 teenagers; e, reported difficulties making friends or interacting with peers. When cells are merged, data specific to each category of HIE is not available but data is reported for 2 HIE subtypes combined. HIE, hypoxic-ischemic encephalopathy; SD, standard deviation; NA, not applicable; ASD, Autism Spectrum Disorder (diagnosis was made using criteria of the Diagnostic Statistical Manual, 4th edition); SB, score obtained from the Stanford-Binet Intelligence Scale, with 100 being the average score; GCI, McCarthy General Cognitive Index, GCI score indicated severe cognitive impairment; WISC, Wechsler Intelligence Scale for Children-III, with 100 being the average score; CBCL, Child Behavior Check List.
Mechanisms of action in therapeutic hypothermia
To fully investigate different aspects of HIE injury, a combination of large (rhesus monkey, pig, fetal sheep) and small (rodent) animal models have been employed to better understand processes of cell death, inflammation, and screening for potential therapies. These have been extensively reviewed (80-83). Paired with clinical data, pre-clinical models provide essential insights into the biochemical mechanisms behind hypoxic injury in term neonates.
Hypothermia can reduce the metabolic demand of cells, with species specific ranges (84) TH makes cells more tolerant of the low oxygen and glucose levels that occur during HIE (85-88). In post-natal rats, hypothermia was found to be neuroprotective via reductions in glycolysis, amino-acid catabolism, and ketolysis (89). TH also has significant anti-inflammatory effects by reducing cytokine production (90,91), microglial activation, and neutrophil recruitment into the injured brain (92,93). Many of these effects are complementary, for example lowering the metabolic demand also helps prevent activation of NMDA receptors (94,95) and the generation of free radicles and ROS, thus protecting lipoprotein membrane integrity (96-98). Furthermore, by reducing inflammation, hypothermia also helps maintain BBB integrity (63,64). The efficacy of TH is also associated with decreased caspase activity and suppressed microglial activation (32,99,100). Recent studies have found that microglia drive injury-induced inflammatory responses in a sex-specific manner, with differences in the microglial number, morphology, migration, and phagocytic activity (101,102). In mouse and rat models of HIE, male mice are more vulnerable to poor acute and long-term developmental outcomes (103-106). To investigate the specific role of microglia in HIE, a genetic microglial depletion model was utilized in early postnatal life in mice (Cx3cr1CreER/+Rosa26DTA/+model). Microglial depletion worsened neuronal damage after HI injury, an effect that was only seen in males (107). Significantly lower IL-10 and TGF-β levels were seen in both male and female pups after microglial depletion. This work suggests that resident microglia play a neuroprotective role early after HIE, but the beneficial effects may be limited to males. These effects highlight the importance of including sex as a biological variable in pre-clinical and clinical studies (108).
Another important variable in neuroprotection after brain injury is time (37,109), as TH is most efficacious at preventing injury during the secondary energy failure (30,32,110,111). A study in fetal sheep found greater oligodendrocyte survival and increased myelin density 5 days after HIE if TH was initiated 2 hours after injury. However, a delay to 5.5 hours resulted in a complete loss of significant oligodendrocyte protection (32). Additional factors to consider for the application of TH are the depth and duration of cooling. Across multiple species, there are windows of temperature reduction that are beneficial (112). A study with neonatal rodents found that temperature up to a 3.5 ℃ reduction yielded neuroprotection after moderate injury (113). Similarly, a study with neonatal pigs found a therapeutic window between a 3.5 to a 5 ℃ reduction, whereas a 8.5 ℃ reduction was detrimental (114). While the exact timing and temperature ranges are species specific, together these results provided important insight into what ranges would be tolerated in humans (115,116). In neonates, cooling durations shorter or longer than 72 hours, or deeper cooling (by >5 ℃), resulted in reduced neuroprotection (35,117). The preclinical studies presented above, and other rigorous translational work have mapped out the boundaries for the effective application of TH in clinical trials for neonates with HIE.
Clinical outcomes in the therapeutic hypothermia era
The NICHD (21), TOBY (34), and the CoolCap (33) trials were randomized clinical trials that expanded upon earlier translational research and clinical studies investigating the potential of TH for treating HIE. These trials implemented strict inclusion criteria to select patients for TH treatment, which was a notable improvement over studies conducted prior to the introduction of cooling. The results of these trials were instrumental in establishing TH as the standard of care for treating HIE in term infants. In a seminal paper, Shankaran et al., reported the first randomized trial for whole body TH from the Members of the NICHD Neonatal Research Network. In this study, 102 infants were assigned to the treatment arm while 106 were assigned to standard supportive care (control group) (21). Overall death or moderate or severe disability occurred in 44% in the TH group versus 62% in the control group (P=0.01), while mortality rate was 24% for the TH group versus 37% in the control group (P=0.08). Neurodevelopmental outcomes were assessed between 18 to 22 months of age. In the hypothermia and control groups, respectively, there was no statistical difference in the rates of cerebral palsy (19% vs. 30%), rates of blindness (7% vs. 14%), and the rates of hearing impairment requiring aids (4% vs. 6%) (21). In a follow-up study, Shankaran et al. reported outcomes for the NICHD trial at 6–7 years of age. Primary outcome data was available for 97 patients in the TH group and for 93 patients in the control group. The study found that death occurred in 27 (28%) and 41 (44%) of the TH and control groups, respectively (P=0.04), while death or severe disability occurred in 38 (41%) and 53 (60%) of the TH and control groups, respectively (P=0.03). However, there was no difference in moderate or severe disability (when disability was assessed without considering mortality), attention-deficit/hyperactivity disorder, or visuospatial dysfunction between the two groups (22). The authors concluded that infants undergoing whole-body TH had a lower rate of the combined end point of death or an IQ score of less than 70 at 6 to 7 years of age compared to those receiving usual care, although the differences were not statistically significant. However, hypothermia was found to significantly reduce death rates among the surviving children (22). In contrast, a later meta-analysis that included 11 randomized controlled trials found that TH not only significantly reduced the risk of death but also of disability at 18 to 24 months of age (40). The NICHD trial also provided crucial insights into the clinical predictors of long-term effects at 6–7 years after HIE treatment with TH versus control, based on outcomes at 8 and 22 months of age (22,118). The rates of moderate or severe disability at 18 months were strongly predictive of the same outcomes at 6–7 years of age. Furthermore, the neuroprotective effects of hypothermia for HIE observed at 18 months persisted through childhood, with a significant reduction in death rates and no increase in disability among survivors.
The Total Body Hypothermia for Neonatal Encephalopathy Trial (TOBY) was a large, randomized, controlled trial of hypothermia for term infants with HIE. At 18 months, children who had been treated with hypothermia had reduced risks of cerebral palsy and improved scores on the Mental Developmental Index and Psychomotor Developmental Index of the Bayley Scales of Infant Development II (BSID-II) and on the Gross Motor Function Classification System (119). The TOBY trial also assessed neurocognitive function at 6–7 years of age with the primary outcome of survival with IQ ≥85. Data on outcomes were available for 280 of the 325 infants who were initially enrolled, with 45 patients being lost to follow-up. The primary outcome occurred among 75 of 145 children (52%) in the hypothermia group vs. 52 of 132 (39%) in the control group. More children in the hypothermia group survived without neurological abnormalities and with better motor-function scores, and rates of CP and moderate or severe disability were lower compared to the control group (all P<0.05). This study showed that moderate hypothermia after perinatal asphyxia resulted in improved neurocognitive outcomes in middle childhood (120).
The CoolCap was another pivotal multicenter randomized controlled trial that assessed if selective head cooling, which differs from the whole-body cooling method used in the NICHD and TOBY trials, could improve neurodevelopmental outcome after HIE (33,121). It also determined if neurodevelopmental outcomes at 18–22 months predicted functional outcomes at 7–8 years of age among surviving children. In this study 234 term infants with moderate to severe neonatal encephalopathy and abnormal amplitude integrated electroencephalography (aEEG) were randomly assigned to either head cooling (n=116), or conventional care (n=118). Data analysis at 18 months revealed that head cooling had no effect in infants with the most severe aEEG changes (P=0.51) but was beneficial in infants with less severe aEEG changes (P=0.009) (33). The authors used the Functional Independence Measure for Children (WeeFIM) as an assessment tool. This scale measures a child’s functional ability in self-care, mobility, and cognition. Qualitative assessment of WeeFIM through phone interviews with parents showed that disability status at 18 months was strongly associated with WeeFIM ratings (P<0.001) indicating that functional outcome at 7–8 years is associated with 18-month neurodevelopmental assessment (39).
To summarize the evidence behind the neuroprotective effect of TH, a meta-analysis of 7 trials, representing 1,214 newborn infants, found that TH reduced the risk of the composite outcome of death or major neurodevelopmental disability at age 18 months by 24% (122). In cases of mild HIE, it is uncertain if children have worse outcomes than those who did not receive TH, highlighting the need for additional adjunctive therapies after HIE (41).
Adjunctive neuroprotective treatments for hypoxic ischemic encephalopathy
While TH is the standard of treatment and it is clearly beneficial, neonates with HIE still experience unacceptably high rate of complications (122), as reviewed above. Therefore, adjunctive therapies to further improve outcomes are urgently needed. A few studies have explored the expanding collection of preclinical and clinical research on HIE neuroprotective treatments used in combination with or without TH (123-130). Some of these therapies, though not an exhaustive list, include noble gases (131), anticonvulsants (132), magnesium (133), cannabidiol (134), progesterone (135), ascorbic acid (136), vitamin E (137), thiamine (138-140), ketogenic diet (141), and other nutraceuticals (142). Aside from adjunctive therapies, other approaches that have shown to improve outcomes in critically ill neonates with HIE are hypocapnia, hypoglycemia, pain control, and functional brain monitoring (143) and remote post ischemic conditioning (144). Interestingly, Sabir et al., recently published the first preclinical multidrug randomized controlled screening trial for nHIE. The authors investigated 25 potential therapeutic agents and reported that caffeine and a sonic hedgehog agonist produced the most beneficial effects. These were followed by allopurinol, melatonin, clemastine, ß-hydroxybutyrate, and omegaven (145). However, results were not reported by sex, despite preclinical evidence suggesting differential responses to therapies based on sex (146,105,147-150). The potential significance of sex differences in response to therapy after HIE has also not been thoroughly investigated in clinical studies, as the majority of studies do not report outcome by sex (151-153). Sex could represent a critical biological variable that might influence treatment strategies for HIE, as suggested by several studies (150,154,155). Here, we will focus on the interventions that have progressed to phase 2 clinical trials or beyond within the past year.
In this context, erythropoietin (EPO) has been the adjunctive neuroprotective treatment most investigated and well characterized since the late 1990s. EPO receptors are found in neurons, glia, microglia, and endothelial cells (156-158), and EPO promotes proliferation and differentiation of neural progenitor cell during brain development (159,160) as well as following hypoxia (161,162). After stroke, oxygen depletion induces expression of hypoxia inducible factor 1α which triggers downstream expression of EPO and its receptors (163,164). EPO provides neuroprotection by reducing apoptotic, inflammatory, and excitotoxic injury (165) in the secondary energy failure and in the tertiary phase of HIE (130), as depicted in Figure 1. The beneficial effects of EPO were extensively studied in preclinical models of HIE with rodents, piglets, and non-human primates, though notably the majority of these studies did not include combined hypothermia (166,167). Next, the pharmacokinetics of EPO in the context of HIE after TH was investigated (168,169). These studies led to phase I and II clinical trials, which demonstrated promising outcomes and an acceptable safety profile (170-172). Two phase 3 randomized placebo-controlled trials (NCT01732146 – not yet reported (173), and NCT02811263) have now been completed. These studies evaluated the effect of intravenous EPO on death and disability at 24 months in term or near-term infants who suffered from moderate or severe HIE (174). The HEAL trial (NCT02811263; study completion September 2022), a multicenter, double-blind, randomized, placebo-controlled trial, investigated the efficacy of intravenous EPO (1,000 U/kg) on postnatal days 1 (<24 h after birth), 2, 3, 4, and 7 on 500 participants. The trial did not result in a lower risk of death or neurodevelopmental impairment than placebo at 2 to 3 years of age (175). There was also no effect of EPO on seizure burden (176) or any effects of EPO treatment on biomarkers of neuroinflammation or brain injury (177). In addition, infants who were administered EPO had a significantly higher likelihood of experiencing thrombosis, and a greater number of serious adverse events were seen in the EPO group compared to those who received placebo (175,178). The authors postulated that since both hypothermia and EPO may activate similar neuroprotective pathways during the acute phase of hypoxic-ischemic injury (179), the use of TH during the trial might have minimized the potential for additional benefits from EPO. Other explanations include potential toxic or suboptimal dosing of EPO or the timing of administration, and the different injury mechanisms in human neonates as compared to preclinical models. Of note, EPO may still be beneficial in low- or middle-income countries where TH is unavailable (180), or in infants with milder forms of HIE (181).
Research on the therapeutic use of allopurinol for HIE began in the late 1990s and early 2000s. Hypoxia leads to an accumulation of hypoxanthine due to ATP depletion. During the reperfusion stage, hypoxanthine is subsequently catabolized by xanthine oxidase into free radicals and superoxide, known to be toxic for the developing brain (124,182). Allopurinol is a xanthine-oxidase inhibitor; thus it acts in the latent and secondary energy failure phase of HIE (129). Several studies in rodents and piglets with and without hypothermia have shown the neuroprotective effects of allopurinol (154,167,183). The pharmacokinetics and pharmacodynamics of allopurinol have been described in neonates with HIE (184). Interestingly, a randomized, double-blind, placebo-controlled multicenter trial reported that maternal allopurinol treatment during suspected fetal hypoxia resulted in significantly lower S100β and neuroketal values, which serve as surrogate measures of brain damage, in the umbilical cord blood exclusively in female neonates. The authors concluded that perinatal maternal treatment with allopurinol may specifically benefit female neonates compared to their male counterparts (185). Interestingly, this sex difference was also seen in earlier preclinical studies, that suggested sex-specific benefits of allopurinol on chronic outcomes after HIE in females (154). Although a meta-analysis of randomized controlled studies investigating the use of allopurinol in newborns with suspected HIE did not find a significant difference in the risk of death or composite outcomes of death and severe neurodevelopmental disability, these studies were too small to rule out the beneficial effect of this intervention (186). An ongoing phase III clinical trial (NCT03162653) aims to recruit 846 neonates to evaluate the effectiveness of allopurinol as an adjunctive treatment to TH (187).
In the early 2000s, studies began investigating the potential neuroprotective effects of melatonin for HIE. Melatonin is a hormone produced by the pineal gland that follows a circadian rhythm. Melatonin acts through receptors, such as MT1, MT2, and MT3, which are highly expressed in the fetal brain and leptomeninges. Its role in brain growth and development has been well established (188-190). Melatonin acts both as a potent scavenger of superoxide anions and stimulates the synthesis of antioxidant enzymes, thereby acting as a direct and indirect antioxidant (191,192). It has neuroprotective effects in HIE by acting in the latent and secondary energy failure phase of HIE (129). It also promotes neuronal and glial development (193,194) and has anti-apoptotic and anti-inflammatory properties (195). Preclinical studies were performed on piglets with and without TH and in lambs without TH with promising results (196). Clinically, there is a need for large, well-designed, and adequately powered randomized clinical trials for the use of melatonin in infants with HIE (197,198). To date, five (one study currently ongoing, NCT02621944) of the six trials performed in term neonates used melatonin’s enteral route of administration but the pharmacokinetic profiles have not been reported (198). The oral bioavailability is less certain in newborns due to higher gastric pH, lower superior mesenteric arterial blood flow and delayed gastric emptying (199). A randomized placebo-controlled trial conducted on infants with moderate-severe HIE (n=25 in each group) investigated the effects of intravenous melatonin (5 mg/kg as 2 h infusion) on postnatal days 1 (<6 h after birth), 2, and 3. The study found that the treatment improved the cognitive composite score but not gross motor function (200).
Studies on the neuroprotective effects of umbilical cord blood (UCB) cells for HIE started as early as the late 1990s. UCB is rich in hematopoietic and mesenchymal stem cells, endothelial progenitor cells, mononuclear cells and growth factors (201). UBC has anti-inflammatory, anti-apoptotic and neurogenerative properties (201,202) by reducing injury during the tertiary phase of neonatal hypoxic injury (130). Further, it has several advantages over other stem cell sources, including easy accessibility, non-invasive banking, lower risk of graft versus host disease, and multi-lineage differentiation potential (203). Thus, UCB intravenous transplantation is a particularly promising approach for the treatment of HIE, with a wide range of potential applications. Several preclinical studies have evaluated the potential of stem cell therapy for HIE. A meta-analysis concluded that about 80% of these studies found a significant improvement of cognitive and/or sensorimotor function, as well as decreased brain damage. That said, only a few have evaluated TH in addition to stem cell transplant (204). A phase 1 clinical study by Cotten et al. (205) showed the feasibility and safety of intravenous infusion of UCB cells for infants with HIE. These findings were confirmed by a more recent phase 1 trial by Tsuji et al. (206). Here, UBC were collected from six newborns with severe HIE, processed, and divided into up to four doses, which were infused at 12–24, 36–48, and 60–72 hours after birth. At 30 days of age, all six infants survived without requiring circulatory or respiratory support, and at 18 months of age, four of the infants had normal neurological development, while two infants had delayed development with cerebral palsy. A phase 2 multi-site double-blinded randomized controlled trial (NCT02612155) was initiated in 2016. However, the study was prematurely stopped after only 35 out of the planned 160 infants were randomized, due to slow enrollment and the logistical complexities of collecting from sick infants, and processing and infusing UCB within the first postnatal hours. The authors performed a pilot phase 1 trial utilizing cryopreserved allogenic mesenchymal stromal cells (MSC) from cord tissue. Six neonates with moderate or severe HIE were enrolled and received one infusion of MSC during TH, 2 infants also received a second dose 2 months later. All infants survived and between the age of 12 and 17 months underwent developmental evaluation, which ranged from average to low-average (207). Although short-term safety signals for UCB cells transplantation have been reassuring, further work is needed to establish the safety and efficacy of cell therapy for brain injury in newborn infants.
Thus far no agent in combination with TH, as compared to TH alone, has demonstrated a statistically significant advantage in terms of mortality and morbidity for neonates with HIE. That said, a recent meta-analysis found that length of hospitalization was significantly reduced when a neuroprotective therapy, such as antioxidants, UBC cells, GABA receptor agonists, NMDA receptor antagonists, neurogenic and angiogenic agents, and glucocorticoids, was used in conjunction with TH, as compared to TH alone (208). In addition, although it is generally agreed that these therapies should be evaluated in combination with TH to determine their potential to improve overall outcomes, investigating their effects as standalone interventions offer a valuable alternative for situations where TH is not feasible or available, such as in low-income settings or when neonates are beyond the optimal window for TH (Table 1). Further, when interventions fail to demonstrate clinical success despite promising preclinical and early clinical data, it is important to consider factors such as optimal dosage timing and method of administration (209), as well as the potential for synergistic effects when combined with other interventions, and how neuroprotective efficacy differs in males and females.
Sex differences can have a significant impact on outcomes after HIE (103,106,150,210,211). Males and females have been shown to exhibit different physiological responses to injury and inflammation in preclinical models of HIE (103,105,212). As such, future studies must consider sex as a biological variable to ensure that both sexes are optimally treated. By accounting for sex differences in HIE, we can potentially identify new targets for therapy and improve outcomes for all patients. As a final note, new treatments for HIE were often developed based on knowledge gained from adult-related conditions (209). However, children are not small adults, as factors like weight, body surface area, and gene expression patterns may not correspond with age (213). Distinctions between newborns and adults have been uncovered in terms of brain injury development. These differences are attributed to variations in brain receptor and enzyme properties, as well as the differing development stages of the liver and kidneys (214), which play a major role in drug clearance.
Conclusions
HIE is one of the leading causes of pediatric death and disability worldwide. TH was a pivotal milestone in improving care for these neonates. However, many patients, even if treated with TH, will develop chronic neurological impairments. Notably, neonates who initially appear neurologically intact after experiencing HIE are still at risk of experiencing cognitive, behavioral, and social impairments later in life. Hence the importance of long-term follow-up for all patients, including those diagnosed with mild HIE, into childhood and adolescence to provide appropriate support, treatment, and academic accommodations as needed. A limitation of this narrative review is that it uses data printed in English, and most data (especially long-term outcomes) are from the USA and resource-rich countries. More research needs to be done investigating long term outcomes of HIE in low-resource settings.
The high personal and social burden caused by HIE, despite TH implementation, calls to the need for adjunctive neuroprotective treatments to improve the outcomes for these neonates. Over the years, several neuroprotective therapies have been explored, and a handful, such as EPO, UCB cells, allopurinol, and melatonin, have advanced to phase 2 clinical trials and beyond. While these treatments have shown promising results in preclinical studies, clinical trials have yet to demonstrate a significant advantage over TH alone. Further research is needed to optimize the dosage, timing, and method of administration of these therapies, while also investigating potential sex differences in their efficacy. While it is crucial to evaluate these therapies in combination with TH to determine their potential to improve overall outcomes, it is also important to investigate the effects of these treatment strategies as standalone interventions as they offer a valuable alternative for situations where TH is not feasible or available. While it would be considered unethical to conduct clinical trials testing neuroprotective treatments without TH in patients who qualify for the standard of care (at centers where TH is available), it is feasible to design clinical trials to evaluate these therapies for neonates who present outside of the therapeutic window for TH. Additionally, research must consider the unique developmental characteristics of neonates, as they are not simply small adults or small children, and they have distinct biological factors that may affect drug response and clearance. Given the remarkable maturation of the neonatal brain and the enduring effects of early brain injury, translational research on treatments that facilitate physiologic conditions for neuroplasticity during development could be particularly effective.
In summary, continued research in the field of neuroprotection for HIE is crucial to improve outcomes for affected neonates. Through the collaboration of scientists, clinicians, medical providers, and families, we can strive to find effective neuroprotective treatments for HIE that will improve the lives of these vulnerable infants.
Acknowledgments
Funding: This work was supported by
Footnote
Provenance and Peer Review: This article was commissioned by the Guest Editor (Antonio F. Corno) for the series “The Impact of the Progresses of Knowledge and Technologies in Pediatrics” published in Translational Pediatrics. The article has undergone external peer review.
Reporting Checklist: The authors have completed the Narrative Review reporting checklist. Available at https://tp.amegroups.com/article/view/10.21037/tp-23-253/rc
Peer Review File: Available at https://tp.amegroups.com/article/view/10.21037/tp-23-253/prf
Conflicts of Interest: All authors have completed the ICMJE uniform disclosure form (available at https://tp.amegroups.com/article/view/10.21037/tp-23-253/coif). The series “The Impact of the Progresses of Knowledge and Technologies in Pediatrics” was commissioned by the editorial office without any funding or sponsorship. The authors have no other conflicts of interest to declare.
Ethical Statement: The authors are accountable for all aspects of the work in ensuring that questions related to the accuracy or integrity of any part of the work are appropriately investigated and resolved.
Open Access Statement: This is an Open Access article distributed in accordance with the Creative Commons Attribution-NonCommercial-NoDerivs 4.0 International License (CC BY-NC-ND 4.0), which permits the non-commercial replication and distribution of the article with the strict proviso that no changes or edits are made and the original work is properly cited (including links to both the formal publication through the relevant DOI and the license). See: https://creativecommons.org/licenses/by-nc-nd/4.0/.
References
- Executive summary: Neonatal encephalopathy and neurologic outcome, second edition. Report of the American College of Obstetricians and Gynecologists’ Task Force on Neonatal Encephalopathy. Obstet Gynecol 2014;123:896-901
- Acun C, Karnati S, Padiyar S, et al. Trends of neonatal hypoxic-ischemic encephalopathy prevalence and associated risk factors in the United States, 2010 to 2018. Am J Obstet Gynecol 2022;S0002-9378(22)00443-4.
- Kurinczuk JJ, White-Koning M, Badawi N. Epidemiology of neonatal encephalopathy and hypoxic-ischaemic encephalopathy. Early Hum Dev 2010;86:329-38. [Crossref] [PubMed]
- McGuire W. Perinatal asphyxia. BMJ Clin Evid 2007;2007:0320.
- Azra Haider B, Bhutta ZA. Birth asphyxia in developing countries: current status and public health implications. Curr Probl Pediatr Adolesc Health Care 2006;36:178-88. [Crossref] [PubMed]
- Tagin M, Abdel-Hady H. Neuroprotection for Perinatal Hypoxic Ischemic Encephalopathy in Low- and Middle-Income Countries. J Pediatr 2015;167:25-8. [Crossref] [PubMed]
- Lawn J, Shibuya K, Stein C. No cry at birth: global estimates of intrapartum stillbirths and intrapartum-related neonatal deaths. Bull World Health Organ 2005;83:409-17.
- Liu L, Johnson HL, Cousens S, et al. Global, regional, and national causes of child mortality: an updated systematic analysis for 2010 with time trends since 2000. Lancet 2012;379:2151-61.
- Namusoke H, Nannyonga MM, Ssebunya R, et al. Incidence and short term outcomes of neonates with hypoxic ischemic encephalopathy in a Peri Urban teaching hospital, Uganda: a prospective cohort study. Matern Health Neonatol Perinatol 2018;4:6. [Crossref] [PubMed]
- Lindström K, Lagerroos P, Gillberg C, et al. Teenage outcome after being born at term with moderate neonatal encephalopathy. Pediatr Neurol 2006;35:268-74. [Crossref] [PubMed]
- van Handel M, Swaab H, de Vries LS, et al. Behavioral outcome in children with a history of neonatal encephalopathy following perinatal asphyxia. J Pediatr Psychol 2010;35:286-95. [Crossref] [PubMed]
- Badawi N, Dixon G, Felix JF, et al. Autism following a history of newborn encephalopathy: more than a coincidence? Dev Med Child Neurol 2006;48:85-9. [Crossref] [PubMed]
- Abate BB, Bimerew M, Gebremichael B, et al. Effects of therapeutic hypothermia on death among asphyxiated neonates with hypoxic-ischemic encephalopathy: A systematic review and meta-analysis of randomized control trials. PLoS One 2021;16:e0247229. [Crossref] [PubMed]
- Sarnat HB, Sarnat MS. Neonatal encephalopathy following fetal distress. A clinical and electroencephalographic study. Arch Neurol 1976;33:696-705. [Crossref] [PubMed]
- Thompson CM, Puterman AS, Linley LL, et al. The value of a scoring system for hypoxic ischaemic encephalopathy in predicting neurodevelopmental outcome. Acta Paediatr 1997;86:757-61. [Crossref] [PubMed]
- Pavageau L, Sánchez PJ, Steven Brown L, et al. Inter-rater reliability of the modified Sarnat examination in preterm infants at 32-36 weeks' gestation. Pediatr Res 2020;87:697-702. [Crossref] [PubMed]
- Chiang F, Castillo M. Hypoxic–Ischemic Encephalopathy (Preterm, Term, and Adult). In: Saba L, Raz E, editors. Neurovascular Imaging: From Basics to Advanced Concepts. New York, NY: Springer; 2016. p. 1-29.
- Gopagondanahalli KR, Li J, Fahey MC, et al. Preterm Hypoxic-Ischemic Encephalopathy. Front Pediatr 2016;4:114. [Crossref] [PubMed]
- Laptook AR. Birth Asphyxia and Hypoxic-Ischemic Brain Injury in the Preterm Infant. Clin Perinatol 2016;43:529-45. [Crossref] [PubMed]
- Ristovska S, Stomnaroska O, Danilovski D. Hypoxic Ischemic Encephalopathy (HIE) in Term and Preterm Infants. Pril 2022;43:77-84. (Makedon Akad Nauk Umet Odd Med Nauki). [Crossref] [PubMed]
- Shankaran S, Laptook AR, Ehrenkranz RA, et al. Whole-body hypothermia for neonates with hypoxic-ischemic encephalopathy. N Engl J Med 2005;353:1574-84. [Crossref] [PubMed]
- Shankaran S, Pappas A, McDonald SA, et al. Childhood outcomes after hypothermia for neonatal encephalopathy. N Engl J Med 2012;366:2085-92. [Crossref] [PubMed]
- Debillon T, Bednarek N, Ego A, et al. LyTONEPAL: long term outcome of neonatal hypoxic encephalopathy in the era of neuroprotective treatment with hypothermia: a French population-based cohort. BMC Pediatr 2018;18:255. [Crossref] [PubMed]
- Lee AC, Kozuki N, Blencowe H, et al. Intrapartum-related neonatal encephalopathy incidence and impairment at regional and global levels for 2010 with trends from 1990. Pediatr Res 2013;74:50-72. [Crossref] [PubMed]
- Volpe JJ. Neonatal encephalopathy: an inadequate term for hypoxic-ischemic encephalopathy. Ann Neurol 2012;72:156-66. [Crossref] [PubMed]
- Russ JB, Simmons R, Glass HC. Neonatal Encephalopathy: Beyond Hypoxic-Ischemic Encephalopathy. Neoreviews 2021;22:e148-62. [Crossref] [PubMed]
- Antonucci R, Porcella A, Pilloni MD. Perinatal asphyxia in the term newborn. Journal of Pediatric and Neonatal Individualized Medicine 2014;3:e030269. (JPNIM).
- van Rijt WJ, Koolhaas GD, Bekhof J, et al. Inborn Errors of Metabolism That Cause Sudden Infant Death: A Systematic Review with Implications for Population Neonatal Screening Programmes. Neonatology 2016;109:297-302. [Crossref] [PubMed]
- French CE, Delon I, Dolling H, et al. Whole genome sequencing reveals that genetic conditions are frequent in intensively ill children. Intensive Care Med 2019;45:627-36.
- Gunn AJ, Gunn TR, de Haan HH, et al. Dramatic neuronal rescue with prolonged selective head cooling after ischemia in fetal lambs. J Clin Invest 1997;99:248-56. [Crossref] [PubMed]
- Bennet L, Rossenrode S, Gunning MI, et al. The cardiovascular and cerebrovascular responses of the immature fetal sheep to acute umbilical cord occlusion. J Physiol 1999;517:247-57. [Crossref] [PubMed]
- Roelfsema V, Bennet L, George S, et al. Window of opportunity of cerebral hypothermia for postischemic white matter injury in the near-term fetal sheep. J Cereb Blood Flow Metab 2004;24:877-86.
- Gluckman PD, Wyatt JS, Azzopardi D, et al. Selective head cooling with mild systemic hypothermia after neonatal encephalopathy: multicentre randomised trial. Lancet 2005;365:663-70. [Crossref] [PubMed]
- Azzopardi D, Brocklehurst P, Edwards D, et al. The TOBY Study. Whole body hypothermia for the treatment of perinatal asphyxial encephalopathy: a randomised controlled trial. BMC Pediatr 2008;8:17. [Crossref] [PubMed]
- Shankaran S, Laptook AR, Pappas A, et al. Effect of Depth and Duration of Cooling on Death or Disability at Age 18 Months Among Neonates With Hypoxic-Ischemic Encephalopathy: A Randomized Clinical Trial. JAMA 2017;318:57-67. [Crossref] [PubMed]
- Natarajan G, Pappas A, Shankaran S. Outcomes in childhood following therapeutic hypothermia for neonatal hypoxic-ischemic encephalopathy (HIE). Semin Perinatol 2016;40:549-55. [Crossref] [PubMed]
- Laptook AR, Shankaran S, Tyson JE, et al. Effect of Therapeutic Hypothermia Initiated After 6 Hours of Age on Death or Disability Among Newborns With Hypoxic-Ischemic Encephalopathy: A Randomized Clinical Trial. JAMA 2017;318:1550-60. [Crossref] [PubMed]
- El-Dib M, Inder TE, Chalak LF, et al. Should therapeutic hypothermia be offered to babies with mild neonatal encephalopathy in the first 6 h after birth? Pediatr Res 2019;85:442-8. [Crossref] [PubMed]
- Guillet R, Edwards AD, Thoresen M, et al. Seven- to eight-year follow-up of the CoolCap trial of head cooling for neonatal encephalopathy. Pediatr Res 2012;71:205-9. [Crossref] [PubMed]
- Jacobs SE, Berg M, Hunt R, et al. Cooling for newborns with hypoxic ischaemic encephalopathy. Cochrane Database Syst Rev 2013;2013:CD003311. [Crossref] [PubMed]
- Saw CL, Rakshasbhuvankar A, Rao S, et al. Current Practice of Therapeutic Hypothermia for Mild Hypoxic Ischemic Encephalopathy. J Child Neurol 2019;34:402-9. [Crossref] [PubMed]
- Bonifacio S. The TIME STUDY: A Randomized Controlled Trial of Therapeutic Hypothermia for Infants With Mild Encephalopathy in California. clinicaltrials.gov; 2020 Mar. Report No.: NCT04176471.
- Thayyil S, Oliveira V, Lally PJ, et al. Hypothermia for encephalopathy in low and middle-income countries (HELIX): study protocol for a randomised controlled trial. Trials 2017;18:432. [Crossref] [PubMed]
- Thayyil S, Pant S, Montaldo P, et al. Hypothermia for moderate or severe neonatal encephalopathy in low-income and middle-income countries (HELIX): a randomised controlled trial in India, Sri Lanka, and Bangladesh. Lancet Glob Health 2021;9:e1273-85. [Crossref] [PubMed]
- Thayyil S, Montaldo P, Krishnan V, et al. Whole-Body Hypothermia, Cerebral Magnetic Resonance Biomarkers, and Outcomes in Neonates With Moderate or Severe Hypoxic-Ischemic Encephalopathy Born at Tertiary Care Centers vs Other Facilities: A Nested Study Within a Randomized Clinical Trial. JAMA Netw Open 2023;6:e2312152. [Crossref] [PubMed]
- Kali GTJ, Pillay S, Pepper MS, et al. Questions about the HELIX trial. Lancet Glob Health 2021;9:e1653. [Crossref] [PubMed]
- Budday S, Steinmann P, Kuhl E. Physical biology of human brain development. Front Cell Neurosci 2015;9:257. [Crossref] [PubMed]
- Swarte R, Lequin M, Cherian P, et al. Imaging patterns of brain injury in term-birth asphyxia. Acta Paediatr 2009;98:586-92. [Crossref] [PubMed]
- Chugani HT, Phelps ME. Maturational changes in cerebral function in infants determined by 18FDG positron emission tomography. Science 1986;231:840-3. [Crossref] [PubMed]
- Chugani HT, Phelps ME, Mazziotta JC. Positron emission tomography study of human brain functional development. Ann Neurol 1987;22:487-97. [Crossref] [PubMed]
- Chugani HT. Review: Metabolic Imaging: A Window on Brain Development and Plasticity. Neuroscientist 1999;5:29-40.
- Chugani HT. Imaging Brain Metabolism in the Newborn. J Child Neurol 2018;33:851-60. [Crossref] [PubMed]
- Li AM, Chau V, Poskitt KJ, et al. White matter injury in term newborns with neonatal encephalopathy. Pediatr Res 2009;65:85-9. [Crossref] [PubMed]
- Piñeiro-Ramos JD, Núñez-Ramiro A, Llorens-Salvador R, et al. Metabolic Phenotypes of Hypoxic-Ischemic Encephalopathy with Normal vs. Pathologic Magnetic Resonance Imaging Outcomes. Metabolites 2020;10:109. [Crossref] [PubMed]
- Kebaya LMN, Kapoor B, Mayorga PC, et al. Subcortical brain volumes in neonatal hypoxic-ischemic encephalopathy. Pediatr Res 2023; Epub ahead of print. [Crossref]
- Chacko A, Andronikou S, Mian A, et al. Cortical ischaemic patterns in term partial-prolonged hypoxic-ischaemic injury-the inter-arterial watershed demonstrated through atrophy, ulegyria and signal change on delayed MRI scans in children with cerebral palsy. Insights Imaging 2020;11:53. [Crossref] [PubMed]
- Shankaran S, McDonald SA, Laptook AR, et al. Neonatal Magnetic Resonance Imaging Pattern of Brain Injury as a Biomarker of Childhood Outcomes following a Trial of Hypothermia for Neonatal Hypoxic-Ischemic Encephalopathy. J Pediatr 2015;167:987-93.e3. [Crossref] [PubMed]
- Parikh NA, Lasky RE, Garza CN, et al. Volumetric and anatomical MRI for hypoxic-ischemic encephalopathy: relationship to hypothermia therapy and neurosensory impairments. J Perinatol 2009;29:143-9. [Crossref] [PubMed]
- Loh WY, Anderson PJ, Cheong JLY, et al. Neonatal basal ganglia and thalamic volumes: very preterm birth and 7-year neurodevelopmental outcomes. Pediatr Res 2017;82:970-8. [Crossref] [PubMed]
- Ogihara T, Hirano K, Ogihara H, et al. Non-protein-bound transition metals and hydroxyl radical generation in cerebrospinal fluid of newborn infants with hypoxic ischemic encephalopathy. Pediatr Res 2003;53:594-9. [Crossref] [PubMed]
- Thornton C, Leaw B, Mallard C, et al. Cell Death in the Developing Brain after Hypoxia-Ischemia. Front Cell Neurosci 2017;11:248. [Crossref] [PubMed]
- Johnston MV. Excitotoxicity in perinatal brain injury. Brain Pathol 2005;15:234-40. [Crossref] [PubMed]
- Kumar A, Mittal R, Khanna HD, et al. Free radical injury and blood-brain barrier permeability in hypoxic-ischemic encephalopathy. Pediatrics 2008;122:e722-7.
- Diaz R, Miguel PM, Deniz BF, et al. Environmental enrichment attenuates the blood brain barrier dysfunction induced by the neonatal hypoxia-ischemia. Int J Dev Neurosci 2016;53:35-45. [Crossref] [PubMed]
- Muramatsu K, Fukuda A, Togari H, et al. Vulnerability to cerebral hypoxic-ischemic insult in neonatal but not in adult rats is in parallel with disruption of the blood-brain barrier. Stroke 1997;28:2281-8; discussion 2288-9. [Crossref] [PubMed]
- Picón-Pagès P, Garcia-Buendia J, Muñoz FJ. Functions and dysfunctions of nitric oxide in brain. Biochim Biophys Acta Mol Basis Dis 2019;1865:1949-67. [Crossref] [PubMed]
- du Plessis AJ, Johnston MV. Hypoxic-ischemic brain injury in the newborn. Cellular mechanisms and potential strategies for neuroprotection. Clin Perinatol 1997;24:627-54.
- Favié LMA, Cox AR, van den Hoogen A, et al. Nitric Oxide Synthase Inhibition as a Neuroprotective Strategy Following Hypoxic-Ischemic Encephalopathy: Evidence From Animal Studies. Front Neurol 2018;9:258. [Crossref] [PubMed]
- Martini S, Austin T, Aceti A, et al. Free radicals and neonatal encephalopathy: mechanisms of injury, biomarkers, and antioxidant treatment perspectives. Pediatr Res 2020;87:823-33. [Crossref] [PubMed]
- Bustelo M, Barkhuizen M, van den Hove DLA, et al. Clinical Implications of Epigenetic Dysregulation in Perinatal Hypoxic-Ischemic Brain Damage. Front Neurol 2020;11:483. [Crossref] [PubMed]
- Robertson C, Finer N. Term infants with hypoxic-ischemic encephalopathy: outcome at 3.5 years. Dev Med Child Neurol 1985;27:473-84. [Crossref] [PubMed]
- Shankaran S, Woldt E, Koepke T, et al. Acute neonatal morbidity and long-term central nervous system sequelae of perinatal asphyxia in term infants. Early Hum Dev 1991;25:135-48. [Crossref] [PubMed]
- Robertson CM. Long-term follow-up of term infants with perinatal asphyxia. In: Stevenson DK, Sunshine P, Benitz WE, editors. Fetal and Neonatal Brain Injury: Mechanisms, Management and the Risks of Practice. 3rd ed. Cambridge: Cambridge University Press; 2003. p. 829-58.
- Marlow N, Rose AS, Rands CE, et al. Neuropsychological and educational problems at school age associated with neonatal encephalopathy. Arch Dis Child Fetal Neonatal Ed 2005;90:F380-7. [Crossref] [PubMed]
- Robertson CM, Perlman M. Follow-up of the term infant after hypoxic-ischemic encephalopathy. Paediatr Child Health 2006;11:278-82.
- WESTIN B. Neonatal asphyxia pallida treated with hypothermia alone or with hypothermia and transfusion of oxygenated blood. Surgery 1959;45:868-79.
- Prec O, Rosenman R, Braun K, et al. The cardiovascular effects of acutely induced hypothermia. J Clin Invest 1949;28:293-300. [Crossref] [PubMed]
- Collins HA, Stahlman M, Scott HW Jr. The occurrence of subcutaneous fat necrosis in an infant following induced hypothermia used as an adjuvant in cardiac surgery. Ann Surg 1953;138:880-5. [Crossref] [PubMed]
- Shankaran S, Laptook A, Wright LL, et al. Whole-body hypothermia for neonatal encephalopathy: animal observations as a basis for a randomized, controlled pilot study in term infants. Pediatrics 2002;110:377-85. [Crossref] [PubMed]
- Koehler RC, Yang ZJ, Lee JK, et al. Perinatal hypoxic-ischemic brain injury in large animal models: Relevance to human neonatal encephalopathy. J Cereb Blood Flow Metab 2018;38:2092-111.
- Jisa KA, Clarey DD, Peeples ES. Magnetic Resonance Imaging Findings of Term and Preterm Hypoxic-Ischemic Encephalopathy: A Review of Relevant Animal Models and Correlation to Human Imaging. Open Neuroimag J 2018;12:55-65. [Crossref] [PubMed]
- Northington FJ. Brief update on animal models of hypoxic-ischemic encephalopathy and neonatal stroke. ILAR J 2006;47:32-8. [Crossref] [PubMed]
- Huang L, Zhao F, Qu Y, et al. Animal models of hypoxic-ischemic encephalopathy: optimal choices for the best outcomes. Rev Neurosci 2017;28:31-43. [Crossref] [PubMed]
- Erecinska M, Thoresen M, Silver IA. Effects of hypothermia on energy metabolism in Mammalian central nervous system. J Cereb Blood Flow Metab 2003;23:513-30. [Crossref] [PubMed]
- Wisnowski JL, Wu TW, Reitman AJ, et al. The effects of therapeutic hypothermia on cerebral metabolism in neonates with hypoxic-ischemic encephalopathy: An in vivo 1H-MR spectroscopy study. J Cereb Blood Flow Metab 2016;36:1075-86. [Crossref] [PubMed]
- Baker AJ, Zornow MH, Grafe MR, et al. Hypothermia prevents ischemia-induced increases in hippocampal glycine concentrations in rabbits. Stroke 1991;22:666-73. [Crossref] [PubMed]
- Busto R, Dietrich WD, Globus MY, et al. Small differences in intraischemic brain temperature critically determine the extent of ischemic neuronal injury. J Cereb Blood Flow Metab 1987;7:729-38. [Crossref] [PubMed]
- Mavroudis CD, Ko T, Volk LE, et al. Does supply meet demand? A comparison of perfusion strategies on cerebral metabolism in a neonatal swine model. J Thorac Cardiovasc Surg 2022;163:e47-58. [Crossref] [PubMed]
- Takenouchi T, Sugiura Y, Morikawa T, et al. Therapeutic hypothermia achieves neuroprotection via a decrease in acetylcholine with a concurrent increase in carnitine in the neonatal hypoxia-ischemia. J Cereb Blood Flow Metab 2015;35:794-805. [Crossref] [PubMed]
- Gibbons H, Sato TA, Dragunow M. Hypothermia suppresses inducible nitric oxide synthase and stimulates cyclooxygenase-2 in lipopolysaccharide stimulated BV-2 cells. Brain Res Mol Brain Res 2003;110:63-75. [Crossref] [PubMed]
- Shah TA, Pallera HK, Kaszowski CL, et al. Therapeutic Hypothermia Inhibits the Classical Complement Pathway in a Rat Model of Neonatal Hypoxic-Ischemic Encephalopathy. Front Neurosci 2021;15:616734. [Crossref] [PubMed]
- Wang GJ, Deng HY, Maier CM, et al. Mild hypothermia reduces ICAM-1 expression, neutrophil infiltration and microglia/monocyte accumulation following experimental stroke. Neuroscience 2002;114:1081-90. [Crossref] [PubMed]
- Inamasu J, Suga S, Sato S, et al. Post-ischemic hypothermia delayed neutrophil accumulation and microglial activation following transient focal ischemia in rats. J Neuroimmunol 2000;109:66-74. [Crossref] [PubMed]
- Mokrushin AA, Pavlinova LI, Borovikov SE. Influence of cooling rate on activity of ionotropic glutamate receptors in brain slices at hypothermia. J Therm Biol 2014;44:5-13. [Crossref] [PubMed]
- Zhang X, Peng K, Zhang X. The Function of the NMDA Receptor in Hypoxic-Ischemic Encephalopathy. Front Neurosci 2020;14:567665. [Crossref] [PubMed]
- Sakr M, Balasundaram P. Neonatal Therapeutic Hypothermia. In: StatPearls. Treasure Island (FL): StatPearls Publishing; March 8, 2023.
- Zhou T, Mo J, Xu W, et al. Mild hypothermia alleviates oxygen-glucose deprivation/reperfusion-induced apoptosis by inhibiting ROS generation, improving mitochondrial dysfunction and regulating DNA damage repair pathway in PC12 cells. Apoptosis 2023;28:447-57. [Crossref] [PubMed]
- Ranjan R. Hypothermic preconditioning attenuates hypobaric hypoxia induced spatial memory impairment in rats. Behav Brain Res 2022;416:113568. [Crossref] [PubMed]
- Wassink G, Davidson JO, Lear CA, et al. A working model for hypothermic neuroprotection. J Physiol 2018;596:5641-54. [Crossref] [PubMed]
- Seitz M, Köster C, Dzietko M, et al. Hypothermia modulates myeloid cell polarization in neonatal hypoxic-ischemic brain injury. J Neuroinflammation 2021;18:266. [Crossref] [PubMed]
- Schwarz JM, Sholar PW, Bilbo SD. Sex differences in microglial colonization of the developing rat brain. J Neurochem 2012;120:948-63. [Crossref] [PubMed]
- Weinhard L, Neniskyte U, Vadisiute A, et al. Sexual dimorphism of microglia and synapses during mouse postnatal development. Dev Neurobiol 2018;78:618-26. [Crossref] [PubMed]
- Mirza MA, Ritzel R, Xu Y, et al. Sexually dimorphic outcomes and inflammatory responses in hypoxic-ischemic encephalopathy. J Neuroinflammation 2015;12:32. [Crossref] [PubMed]
- Cohen SS, Stonestreet BS. Sex differences in behavioral outcome following neonatal hypoxia ischemia: Insights from a clinical meta-analysis and a rodent model of induced hypoxic ischemic injury. Exp Neurol 2014;256:70-3. [Crossref] [PubMed]
- Hill CA, Fitch RH. Sex differences in mechanisms and outcome of neonatal hypoxia-ischemia in rodent models: implications for sex-specific neuroprotection in clinical neonatal practice. Neurol Res Int 2012;2012:867531. [Crossref] [PubMed]
- Smith AL, Alexander M, Rosenkrantz TS, et al. Sex differences in behavioral outcome following neonatal hypoxia ischemia: insights from a clinical meta-analysis and a rodent model of induced hypoxic ischemic brain injury. Exp Neurol 2014;254:54-67. [Crossref] [PubMed]
- Tsuji S, Di Martino E, Mukai T, et al. Aggravated brain injury after neonatal hypoxic ischemia in microglia-depleted mice. J Neuroinflammation 2020;17:111. [Crossref] [PubMed]
- Mauvais-Jarvis F, Bairey Merz N, Barnes PJ, et al. Sex and gender: modifiers of health, disease, and medicine. Lancet 2020;396:565-82. [Crossref] [PubMed]
- Thoresen M, Tooley J, Liu X, et al. Time is brain: starting therapeutic hypothermia within three hours after birth improves motor outcome in asphyxiated newborns. Neonatology 2013;104:228-33. [Crossref] [PubMed]
- Thoresen M, Penrice J, Lorek A, et al. Mild hypothermia after severe transient hypoxia-ischemia ameliorates delayed cerebral energy failure in the newborn piglet. Pediatr Res 1995;37:667-70. [Crossref] [PubMed]
- Gunn AJ, Bennet L, Gunning MI, et al. Cerebral hypothermia is not neuroprotective when started after postischemic seizures in fetal sheep. Pediatr Res 1999;46:274-80. [Crossref] [PubMed]
- Sabir H, Bonifacio SL, Gunn AJ, et al. Unanswered questions regarding therapeutic hypothermia for neonates with neonatal encephalopathy. Semin Fetal Neonatal Med 2021;26:101257. [Crossref] [PubMed]
- Wood T, Osredkar D, Puchades M, et al. Treatment temperature and insult severity influence the neuroprotective effects of therapeutic hypothermia. Sci Rep 2016;6:23430. [Crossref] [PubMed]
- Alonso-Alconada D, Broad KD, Bainbridge A, et al. Brain cell death is reduced with cooling by 3.5°C to 5°C but increased with cooling by 8.5°C in a piglet asphyxia model. Stroke 2015;46:275-8. [Crossref] [PubMed]
- Davidson JO, Wassink G, Yuill CA, et al. How long is too long for cerebral cooling after ischemia in fetal sheep? J Cereb Blood Flow Metab 2015;35:751-8. [Crossref] [PubMed]
- Davidson JO, Draghi V, Whitham S, et al. How long is sufficient for optimal neuroprotection with cerebral cooling after ischemia in fetal sheep? J Cereb Blood Flow Metab 2018;38:1047-59.
- Shankaran S, Laptook AR, Pappas A, et al. Effect of depth and duration of cooling on deaths in the NICU among neonates with hypoxic ischemic encephalopathy: a randomized clinical trial. JAMA 2014;312:2629-39. [Crossref] [PubMed]
- Pappas A, Shankaran S, McDonald SA, et al. Cognitive outcomes after neonatal encephalopathy. Pediatrics 2015;135:e624-34. [Crossref] [PubMed]
- Azzopardi DV, Strohm B, Edwards AD, et al. Moderate hypothermia to treat perinatal asphyxial encephalopathy. N Engl J Med 2009;361:1349-58. [Crossref] [PubMed]
- Azzopardi D, Strohm B, Marlow N, et al. Effects of hypothermia for perinatal asphyxia on childhood outcomes. N Engl J Med 2014;371:140-9. [Crossref] [PubMed]
- Wyatt JS, Gluckman PD, Liu PY, et al. Determinants of outcomes after head cooling for neonatal encephalopathy. Pediatrics 2007;119:912-21. [Crossref] [PubMed]
- Tagin MA, Woolcott CG, Vincer MJ, et al. Hypothermia for neonatal hypoxic ischemic encephalopathy: an updated systematic review and meta-analysis. Arch Pediatr Adolesc Med 2012;166:558-66. [Crossref] [PubMed]
- Robertson NJ, Tan S, Groenendaal F, et al. Which neuroprotective agents are ready for bench to bedside translation in the newborn infant? J Pediatr 2012;160:544-552.e4. [Crossref] [PubMed]
- Bel Fv, Groenendaal F. Drugs for neuroprotection after birth asphyxia: Pharmacologic adjuncts to hypothermia. Semin Perinatol 2016;40:152-9. [Crossref] [PubMed]
- Albrecht M, Zitta K, Groenendaal F, et al. Neuroprotective strategies following perinatal hypoxia-ischemia: Taking aim at NOS. Free Radic Biol Med 2019;142:123-31. [Crossref] [PubMed]
- Nair J, Kumar VHS. Current and Emerging Therapies in the Management of Hypoxic Ischemic Encephalopathy in Neonates. Children (Basel) 2018;5:99. [Crossref] [PubMed]
- Wang Q, Lv H, Lu L, et al. Neonatal hypoxic-ischemic encephalopathy: emerging therapeutic strategies based on pathophysiologic phases of the injury. J Matern Fetal Neonatal Med 2019;32:3685-92. [Crossref] [PubMed]
- Solevåg AL, Schmölzer GM, Cheung PY. Novel interventions to reduce oxidative-stress related brain injury in neonatal asphyxia. Free Radic Biol Med 2019;142:113-22. [Crossref] [PubMed]
- Chakkarapani AA, Aly H, Benders M, et al. Therapies for neonatal encephalopathy: Targeting the latent, secondary and tertiary phases of evolving brain injury. Semin Fetal Neonatal Med 2021;26:101256. [Crossref] [PubMed]
- Molloy EJ, El-Dib M, Juul SE, et al. Neuroprotective therapies in the NICU in term infants: present and future. Pediatr Res 2023;93:1819-27. [Crossref] [PubMed]
- Yin H, Chen Z, Zhao H, et al. Noble gas and neuroprotection: From bench to bedside. Front Pharmacol 2022;13:1028688. [Crossref] [PubMed]
- Nuñez-Ramiro A, Benavente-Fernández I, Valverde E, et al. Topiramate plus Cooling for Hypoxic-Ischemic Encephalopathy: A Randomized, Controlled, Multicenter, Double-Blinded Trial. Neonatology 2019;116:76-84.
- Lingam I, Robertson NJ. Magnesium as a Neuroprotective Agent: A Review of Its Use in the Fetus, Term Infant with Neonatal Encephalopathy, and the Adult Stroke Patient. Dev Neurosci 2018;40:1-12. [Crossref] [PubMed]
- Martínez-Orgado J, Villa M, Del Pozo A. Cannabidiol for the Treatment of Neonatal Hypoxic-Ischemic Brain Injury. Front Pharmacol 2020;11:584533.
- Lee MT, McNicholas R, Miall L, et al. Progesterone as a Neuroprotective Agent in Neonatal Hypoxic-Ischaemic Encephalopathy: A Systematic Review. Dev Neurosci 2023;45:76-93. [Crossref] [PubMed]
- Kangisser L, Tan E, Bellomo R, et al. Neuroprotective Properties of Vitamin C: A Scoping Review of Pre-Clinical and Clinical Studies. J Neurotrauma 2021;38:2194-205. [Crossref] [PubMed]
- Kolnik S, Wood TR. Role of Vitamin E in Neonatal Neuroprotection: A Comprehensive Narrative Review. Life (Basel) 2022;12:1083. [Crossref] [PubMed]
- Sechi GP, Bardanzellu F, Pintus MC, et al. Thiamine as a Possible Neuroprotective Strategy in Neonatal Hypoxic-Ischemic Encephalopathy. Antioxidants (Basel) 2021;11:42. [Crossref] [PubMed]
- Sechi G, Sechi MM. New Therapeutic Paradigms in Neonatal Hypoxic-Ischemic Encephalopathy. ACS Chem Neurosci 2023;14:1004-6. [Crossref] [PubMed]
- Islas-Fabila P, Bonilla-Jaime H, González-Hernández M, et al. Effect of thiamine pyrophosphate on the characteristics of farrowing and piglet vitality. Theriogenology 2023;200:49-59. [Crossref] [PubMed]
- Zhou Y, Sun L, Wang H. Ketogenic Diet for Neonatal Hypoxic-Ischemic Encephalopathy. ACS Chem Neurosci 2023;14:1-8. [Crossref] [PubMed]
- Reyes-Corral M, Sola-Idígora N, de la Puerta R, et al. Nutraceuticals in the Prevention of Neonatal Hypoxia-Ischemia: A Comprehensive Review of their Neuroprotective Properties, Mechanisms of Action and Future Directions. Int J Mol Sci 2021;22:2524. [Crossref] [PubMed]
- Cannavò L, Perrone S, Gitto E. Brain-Oriented Strategies for Neuroprotection of Asphyxiated Newborns in the First Hours of Life. Pediatr Neurol 2023;143:44-9. [Crossref] [PubMed]
- Hassell KJ, Ezzati M, Alonso-Alconada D, et al. New horizons for newborn brain protection: enhancing endogenous neuroprotection. Arch Dis Child Fetal Neonatal Ed 2015;100:F541-52. [Crossref] [PubMed]
- Sabir H, Maes E, Zweyer M, et al. Comparing the efficacy in reducing brain injury of different neuroprotective agents following neonatal hypoxia-ischemia in newborn rats: a multi-drug randomized controlled screening trial. Sci Rep 2023;13:9467. [Crossref] [PubMed]
- Hill CA, Threlkeld SW, Fitch RH. Early testosterone modulated sex differences in behavioral outcome following neonatal hypoxia ischemia in rats. Int J Dev Neurosci 2011;29:381-8. [Crossref] [PubMed]
- Smith AL, Rosenkrantz TS, Fitch RH. Effects of Sex and Mild Intrainsult Hypothermia on Neuropathology and Neural Reorganization following Neonatal Hypoxic Ischemic Brain Injury in Rats. Neural Plast 2016;2016:2585230. [Crossref] [PubMed]
- Brandt MJV, Nijboer CH, Nessel I, et al. Nutritional Supplementation Reduces Lesion Size and Neuroinflammation in a Sex-Dependent Manner in a Mouse Model of Perinatal Hypoxic-Ischemic Brain Injury. Nutrients 2021;14:176. [Crossref] [PubMed]
- Kremsky I, Ma Q, Li B, et al. Fetal hypoxia results in sex- and cell type-specific alterations in neonatal transcription in rat oligodendrocyte precursor cells, microglia, neurons, and oligodendrocytes. Cell Biosci 2023;13:58. [Crossref] [PubMed]
- Saadat A, Blackwell A, Kaszowski C, et al. Therapeutic hypothermia demonstrates sex-dependent improvements in motor function in a rat model of neonatal hypoxic ischemic encephalopathy. Behav Brain Res 2023;437:114119. [Crossref] [PubMed]
- Rosenkrantz TS, Hussain Z, Fitch RH. Sex Differences in Brain Injury and Repair in Newborn Infants: Clinical Evidence and Biological Mechanisms. Front Pediatr 2019;7:211. [Crossref] [PubMed]
- Zhou KQ, Davidson JO, Gunn AJ. Does sex materially modulate responses to therapeutic hypothermia? Pediatr Res 2023; Epub ahead of print. [Crossref]
- Sewell EK, Shankaran S, Natarajan G, et al. Evaluation of heterogeneity in effect of therapeutic hypothermia by sex among infants with neonatal encephalopathy. Pediatr Res 2023; Epub ahead of print. [Crossref]
- Rodríguez-Fanjul J, Durán Fernández-Feijóo C, Lopez-Abad M, et al. Neuroprotection with hypothermia and allopurinol in an animal model of hypoxic-ischemic injury: Is it a gender question? PLoS One 2017;12:e0184643. [Crossref] [PubMed]
- McLeod R, Rosenkrantz T, Fitch RH. Therapeutic Interventions in Rat Models of Preterm Hypoxic Ischemic Injury: Effects of Hypothermia, Caffeine, and the Influence of Sex. Life (Basel) 2022;12:1514. [Crossref] [PubMed]
- Buemi M, Cavallaro E, Floccari F, et al. The pleiotropic effects of erythropoietin in the central nervous system. J Neuropathol Exp Neurol 2003;62:228-36.
- Nagai A, Nakagawa E, Choi HB, et al. Erythropoietin and erythropoietin receptors in human CNS neurons, astrocytes, microglia, and oligodendrocytes grown in culture. J Neuropathol Exp Neurol 2001;60:386-92. [Crossref] [PubMed]
- Yamaji R, Okada T, Moriya M, et al. Brain capillary endothelial cells express two forms of erythropoietin receptor mRNA. Eur J Biochem 1996;239:494-500. [Crossref] [PubMed]
- Yu X, Shacka JJ, Eells JB, et al. Erythropoietin receptor signalling is required for normal brain development. Development 2002;129:505-16. [Crossref] [PubMed]
- Chen ZY, Asavaritikrai P, Prchal JT, et al. Endogenous erythropoietin signaling is required for normal neural progenitor cell proliferation. J Biol Chem 2007;282:25875-83. [Crossref] [PubMed]
- Gonzalez FF, Larpthaveesarp A, McQuillen P, et al. Erythropoietin increases neurogenesis and oligodendrogliosis of subventricular zone precursor cells after neonatal stroke. Stroke 2013;44:753-8. [Crossref] [PubMed]
- Gonzalez FF, McQuillen P, Mu D, et al. Erythropoietin enhances long-term neuroprotection and neurogenesis in neonatal stroke. Dev Neurosci 2007;29:321-30. [Crossref] [PubMed]
- Ikeda E. Cellular response to tissue hypoxia and its involvement in disease progression. Pathol Int 2005;55:603-10. [Crossref] [PubMed]
- Li J, Tao T, Xu J, et al. HIF 1α attenuates neuronal apoptosis by upregulating EPO expression following cerebral ischemia reperfusion injury in a rat MCAO model. Int J Mol Med 2020;45:1027-36.
- Villa P, van Beek J, Larsen AK, et al. Reduced functional deficits, neuroinflammation, and secondary tissue damage after treatment of stroke by nonerythropoietic erythropoietin derivatives. J Cereb Blood Flow Metab 2007;27:552-63. [Crossref] [PubMed]
- Rangarajan V, Juul SE. Erythropoietin: emerging role of erythropoietin in neonatal neuroprotection. Pediatr Neurol 2014;51:481-8. [Crossref] [PubMed]
- Victor S, Rocha-Ferreira E, Rahim A, et al. New possibilities for neuroprotection in neonatal hypoxic-ischemic encephalopathy. Eur J Pediatr 2022;181:875-87. [Crossref] [PubMed]
- Frymoyer A, Juul SE, Massaro AN, et al. High-dose erythropoietin population pharmacokinetics in neonates with hypoxic-ischemic encephalopathy receiving hypothermia. Pediatr Res 2017;81:865-72.
- Wu YW, Bauer LA, Ballard RA, et al. Erythropoietin for neuroprotection in neonatal encephalopathy: safety and pharmacokinetics. Pediatrics 2012;130:683-91. [Crossref] [PubMed]
- Elmahdy H, El-Mashad AR, El-Bahrawy H, et al. Human recombinant erythropoietin in asphyxia neonatorum: pilot trial. Pediatrics 2010;125:e1135-42. [Crossref] [PubMed]
- Zhu C, Kang W, Xu F, et al. Erythropoietin improved neurologic outcomes in newborns with hypoxic-ischemic encephalopathy. Pediatrics 2009;124:e218-26. [Crossref] [PubMed]
- Wu YW, Mathur AM, Chang T, et al. High-Dose Erythropoietin and Hypothermia for Hypoxic-Ischemic Encephalopathy: A Phase II Trial. Pediatrics 2016;137:e20160191. [Crossref] [PubMed]
- Oorschot DE, Sizemore RJ, Amer AR. Treatment of Neonatal Hypoxic-Ischemic Encephalopathy with Erythropoietin Alone, and Erythropoietin Combined with Hypothermia: History, Current Status, and Future Research. Int J Mol Sci 2020;21:1487. [Crossref] [PubMed]
- Juul SE, Comstock BA, Heagerty PJ, et al. High-Dose Erythropoietin for Asphyxia and Encephalopathy (HEAL): A Randomized Controlled Trial - Background, Aims, and Study Protocol. Neonatology 2018;113:331-8. [Crossref] [PubMed]
- Wu YW, Comstock BA, Gonzalez FF, et al. Trial of Erythropoietin for Hypoxic-Ischemic Encephalopathy in Newborns. N Engl J Med 2022;387:148-59. [Crossref] [PubMed]
- Glass HC, Wusthoff CJ, Comstock BA, et al. Risk of seizures in neonates with hypoxic-ischemic encephalopathy receiving hypothermia plus erythropoietin or placebo. Pediatr Res 2023;94:252-9. [Crossref] [PubMed]
- Juul SE, Voldal E, Comstock BA, et al. Association of High-Dose Erythropoietin With Circulating Biomarkers and Neurodevelopmental Outcomes Among Neonates With Hypoxic Ischemic Encephalopathy: A Secondary Analysis of the HEAL Randomized Clinical Trial. JAMA Netw Open 2023;6:e2322131. [Crossref] [PubMed]
- Juul SE, Comstock BA, Cornet MC, et al. Safety of High Dose Erythropoietin Used with Therapeutic Hypothermia as Treatment for Newborn Hypoxic-Ischemic Encephalopathy: Secondary Analysis of the HEAL Randomized Controlled Trial. J Pediatr 2023;258:113400. [Crossref] [PubMed]
- Wassink G, Davidson JO, Crisostomo A, et al. Recombinant erythropoietin does not augment hypothermic white matter protection after global cerebral ischaemia in near-term fetal sheep. Brain Commun 2021;3:fcab172. [Crossref] [PubMed]
- Ezenwa B, Ezeaka C, Fajolu I, et al. Impact of Erythropoietin in the management of Hypoxic Ischaemic Encephalopathy in resource-constrained settings: protocol for a randomized control trial. BMC Neurol 2020;20:171. [Crossref] [PubMed]
- DuPont TL, Baserga M, Lowe J, et al. Darbepoetin as a neuroprotective agent in mild neonatal encephalopathy: a randomized, placebo-controlled, feasibility trial. J Perinatol 2021;41:1339-46. [Crossref] [PubMed]
- McCord JM. Oxygen-derived free radicals in postischemic tissue injury. N Engl J Med 1985;312:159-63. [Crossref] [PubMed]
- Durán Fernández-Feijóo C, Rodríguez-Fanjul J, Lopez-Abat M, et al. Effects of Hypothermia and Allopurinol on Oxidative Status in a Rat Model of Hypoxic Ischemic Encephalopathy. Antioxidants (Basel) 2021;10:1523. [Crossref] [PubMed]
- Chu WY, Annink KV, Nijstad AL, et al. Pharmacokinetic/Pharmacodynamic Modelling of Allopurinol, its Active Metabolite Oxypurinol, and Biomarkers Hypoxanthine, Xanthine and Uric Acid in Hypoxic-Ischemic Encephalopathy Neonates. Clin Pharmacokinet 2022;61:321-33. [Crossref] [PubMed]
- Kaandorp JJ, Benders MJ, Schuit E, et al. Maternal allopurinol administration during suspected fetal hypoxia: a novel neuroprotective intervention? A multicentre randomised placebo controlled trial. Arch Dis Child Fetal Neonatal Ed 2015;100:F216-23. [Crossref] [PubMed]
- Chaudhari T, McGuire W. Allopurinol for preventing mortality and morbidity in newborn infants with hypoxic-ischaemic encephalopathy. Cochrane Database Syst Rev 2012;CD006817. [Crossref] [PubMed]
- Maiwald CA, Annink KV, Rüdiger M, et al. Effect of allopurinol in addition to hypothermia treatment in neonates for hypoxic-ischemic brain injury on neurocognitive outcome (ALBINO): study protocol of a blinded randomized placebo-controlled parallel group multicenter trial for superiority (phase III). BMC Pediatr 2019;19:210. [Crossref] [PubMed]
- Sagrillo-Fagundes L, Assunção Salustiano EM, Yen PW, et al. Melatonin in Pregnancy: Effects on Brain Development and CNS Programming Disorders. Curr Pharm Des 2016;22:978-86. [Crossref] [PubMed]
- Tordjman S, Chokron S, Delorme R, et al. Melatonin: Pharmacology, Functions and Therapeutic Benefits. Curr Neuropharmacol 2017;15:434-43. [Crossref] [PubMed]
- Drew JE, Williams LM, Hannah LT, et al. Identification and characterisation of 2-[125I]iodomelatonin binding and Mel1a melatonin receptor expression in the human fetal leptomeninges. Brain Res 1997;761:87-92.
- Okatani Y, Wakatsuki A, Shinohara K, et al. Melatonin stimulates glutathione peroxidase activity in human chorion. J Pineal Res 2001;30:199-205. [Crossref] [PubMed]
- Okatani Y, Wakatsuki A, Kaneda C. Melatonin increases activities of glutathione peroxidase and superoxide dismutase in fetal rat brain. J Pineal Res 2000;28:89-96. [Crossref] [PubMed]
- Moriya T, Horie N, Mitome M, et al. Melatonin influences the proliferative and differentiative activity of neural stem cells. J Pineal Res 2007;42:411-8. [Crossref] [PubMed]
- Sotthibundhu A, Phansuwan-Pujito P, Govitrapong P. Melatonin increases proliferation of cultured neural stem cells obtained from adult mouse subventricular zone. J Pineal Res 2010;49:291-300. [Crossref] [PubMed]
- Chitimus DM, Popescu MR, Voiculescu SE, et al. Melatonin's Impact on Antioxidative and Anti-Inflammatory Reprogramming in Homeostasis and Disease. Biomolecules 2020;10:1211. [Crossref] [PubMed]
- Pang R, Advic-Belltheus A, Meehan C, et al. Melatonin for Neonatal Encephalopathy: From Bench to Bedside. Int J Mol Sci 2021;22:5481. [Crossref] [PubMed]
- Cardinali DP. An Assessment of Melatonin's Therapeutic Value in the Hypoxic-Ischemic Encephalopathy of the Newborn. Front Synaptic Neurosci 2019;11:34. [Crossref] [PubMed]
- Ahmed J, Pullattayil S AK, Robertson NJ, et al. Melatonin for neuroprotection in neonatal encephalopathy: A systematic review & meta-analysis of clinical trials. Eur J Paediatr Neurol 2021;31:38-45.
- Kaye JL. Review of paediatric gastrointestinal physiology data relevant to oral drug delivery. Int J Clin Pharm 2011;33:20-4. [Crossref] [PubMed]
- Jerez-Calero A, Salvatierra-Cuenca MT, Benitez-Feliponi Á, et al. Hypothermia Plus Melatonin in Asphyctic Newborns: A Randomized-Controlled Pilot Study. Pediatr Crit Care Med 2020;21:647-55. [Crossref] [PubMed]
- Xi Y, Yue G, Gao S, et al. Human umbilical cord blood mononuclear cells transplantation for perinatal brain injury. Stem Cell Res Ther 2022;13:458. [Crossref] [PubMed]
- Bruschettini M, Romantsik O, Moreira A, et al. Stem cell-based interventions for the prevention of morbidity and mortality following hypoxic-ischaemic encephalopathy in newborn infants. Cochrane Database Syst Rev 2020;8:CD013202. [Crossref] [PubMed]
- Rallapalli S, Guhathakurta S, Narayan S, et al. Generation of clinical-grade red blood cells from human umbilical cord blood mononuclear cells. Cell Tissue Res 2019;375:437-49. [Crossref] [PubMed]
- Serrenho I, Rosado M, Dinis A, et al. Stem Cell Therapy for Neonatal Hypoxic-Ischemic Encephalopathy: A Systematic Review of Preclinical Studies. Int J Mol Sci 2021;22:3142. [Crossref] [PubMed]
- Cotten CM, Murtha AP, Goldberg RN, et al. Feasibility of autologous cord blood cells for infants with hypoxic-ischemic encephalopathy. J Pediatr 2014;164:973-979.e1. [Crossref] [PubMed]
- Tsuji M, Sawada M, Watabe S, et al. Autologous cord blood cell therapy for neonatal hypoxic-ischaemic encephalopathy: a pilot study for feasibility and safety. Sci Rep 2020;10:4603.
- Cotten CM, Fisher K, Malcolm W, et al. A Pilot Phase I Trial of Allogeneic Umbilical Cord Tissue-Derived Mesenchymal Stromal Cells in Neonates With Hypoxic-Ischemic Encephalopathy. Stem Cells Transl Med 2023;12:355-64. [Crossref] [PubMed]
- Ovcjak A, Pontello R, Miller SP, et al. Hypothermia combined with neuroprotective adjuvants shortens the duration of hospitalization in infants with hypoxic ischemic encephalopathy: Meta-analysis. Front Pharmacol 2022;13:1037131. [Crossref] [PubMed]
- Narayanamurthy R, Yang JJ, Yager JY, et al. Drug delivery platforms for neonatal brain injury. J Control Release 2021;330:765-87. [Crossref] [PubMed]
- Al Mamun A, Yu H, Romana S, et al. Inflammatory Responses are Sex Specific in Chronic Hypoxic-Ischemic Encephalopathy. Cell Transplant 2018;27:1328-39. [Crossref] [PubMed]
- Kumar AJ, Helou AY, Petrucelli BA, et al. Sensorimotor development of male and female rats subjected to neonatal anoxia. Dev Psychobiol 2022;64:e22291. [Crossref] [PubMed]
- Demarest TG, Schuh RA, Waddell J, et al. Sex-dependent mitochondrial respiratory impairment and oxidative stress in a rat model of neonatal hypoxic-ischemic encephalopathy. J Neurochem 2016;137:714-29.
- Leeder JS, Kearns GL, Spielberg SP, et al. Understanding the relative roles of pharmacogenetics and ontogeny in pediatric drug development and regulatory science. J Clin Pharmacol 2010;50:1377-87. [Crossref] [PubMed]
- Rodríguez-Nogales C, González-Fernández Y, Aldaz A, et al. Nanomedicines for Pediatric Cancers. ACS Nano 2018;12:7482-96. [Crossref] [PubMed]