The potential of cardiac xenotransplantation for management of infants with complex congenital heart disease
Introduction
Current status of pediatric cardiac transplantation
Background
Cardiac transplantation is the most effective therapy for treating end-stage heart failure in children. Short and long-term outcomes have reached historical highs. A total of 30 pediatric heart transplants took place in 1984 with a median survival of 3.5 years (1). Today, over 600 children worldwide undergo cardiac transplantation each year with an expected overall median survival of 18 years. Results are age-dependent and superior in patients who undergo transplant at an earlier age. Median survival is 24.5 years in infants compared to 14.3 years for those transplanted between the ages of 11–17. Greater than 60% of infants who survive the first post-transplant year are still alive 25 years later (2).
Despite these excellent results, the annual number of transplants has remained stagnant over the last 10 years. Twenty to thirty percent of children listed for transplant will die on the waitlist each year before a suitable organ can be found (3). The primary reason for this is simple—the need for transplant exceeds the available supply of deceased human donor hearts.
Combating waitlist mortality
Physicians caring for children with heart failure are combating waitlist mortality through three strategic efforts: (I) reducing demand for transplantation; (II) increasing the available supply of donor hearts; and (III) seeking alternatives to safely extend waitlist times. The first of these efforts centers on judicious listing and appropriate prioritization of children waiting for transplant. This is reflected by modifications made in 2016 to the allocation system for pediatric heart transplantation by the Organ Procurement and Transplant Network (4). In addition, stakeholders are advocating to implement risk models to determine transplant candidacy. They argue that limited resources should be protected, and the medical community must reduce the use of cardiac transplant for patients at the highest risk of death within 1 year of transplant (5).
The second effort has providers working to increase the supply of donor hearts either by improving utilization of currently offered organs or seeking alternative methods to increase human donation. Reports indicate that 18–57% of offered hearts are not presently transplanted (6). The International Society for Heart and Lung Transplantation (ISHLT) has spearheaded efforts in conjunction with other groups to define the impact of donor characteristics on transplant outcomes (7-10). They sought to dispel perceived limitations on organ acceptance based on donor characteristics so that fewer hearts offered in donation go un-transplanted.
The community continues to push the boundaries of ABO-incompatible heart transplantation in children. This initiative expands the pool of donors from which any given recipient can receive an organ. Depending on the country, children as old as four are candidates to safely undergo transplantation of a heart from a donor of an incompatible ABO blood group, provided that isohemagglutin titers are below 1:16 (11). Additionally, certain pediatric groups have followed the lead of colleagues working with adults and have renewed their focus on procuring hearts following circulatory death. Limited pediatric data suggest acceptable outcomes, albeit inferior to hearts obtained following brain death (12).
The third effort, i.e., to safely extend waitlist times, centers on the application of technology to improve cardiac output until a suitable organ can be found. Pediatric mechanical circulatory support (MCS) utilization is on the rise worldwide. Thirty-seven percent of children listed for transplant are now bridged to transplant with a ventricular assist device (VAD) and outcomes have improved with each passing year. The most recent Pediatric Interagency Registry for Mechanical Circulatory Support report indicated a positive outcome (alive on device or successfully bridged to transplant/recovery) for 82% of VAD recipients 6 months following implant (13).
However, there remains a disparity in the use of this technology among children with congenital heart disease (CHD) versus those with dilated cardiomyopathy (2). VAD outcomes are inferior for patients with CHD, particularly for those patients with first stage palliated single ventricle disease (13). Despite these efforts, waitlist mortality among neonates and infants remains disproportionately high, warranting further exploration into alternatives.
Cardiac xenotransplantation
Background
Medical professionals have long dreamt that xenotransplantation would be the definitive solution for patients with end-stage heart failure. Transplant pioneer Norman Shumway has often been quoted, “Xenotransplantation is the future of transplantation, and always will be.” This pessimistic sentiment is understandable considering highly publicized failures such as those of Dr. James Hardy and Dr. Leonard Bailey who transplanted nonhuman primate (NHP) hearts into humans who succumbed fairly rapidly (14,15). Advances in molecular biology and genome editing are beginning to shift attitudes once again and encourage physicians to hope that xenotransplantation will soon have clinical utility.
Since the 1990s, scientists have been experimenting with genome editing in pigs for the purpose of producing organs compatible with the human immune system (16). Early molecular biology techniques in addition to lack of identified xenoantigen targets precluded rapid and widespread clinical translation. The development of CRISPR-Cas9 technology (clustered randomly interspaced short palindromic repeats and the associated protein 9) made genome editing easier, quicker, and less expensive (17,18).
In addition, scientists identified three major carbohydrate antigens expressed on pig vascular endothelium against which humans have natural antibodies (Table 1). Genetically engineered “triple knockout” (TKO) pigs that do not express any of these antigens are now available (19). Human infant serum antibody binding to TKO pig cells is non-existent or minimal (Figure 1). Researchers have introduced other genetic modifications, e.g., protective human transgenes, which add further protection against the human immune response (21). This progress has prompted a recent push for clinical application, including one clinical heart transplant in an adult on ‘compassionate’ grounds (22) followed recently by a second.
Table 1
Carbohydrate (abbreviation) | Responsible enzyme | Gene-knockout pig |
---|---|---|
1. Galactose-α1,3-galactose (Gal) | α1,3-galactosyltransferase | GTKO |
2. N-glycolylneuraminic acid (Neu5Gc) | Cytidine monophosphate-N-acetylneuraminic acid hydroxylase (CMAH) | CMAH-KO |
3. Sda | β-1,4N-acetylgalactosaminyltransferase | β4GalNT2-KO |
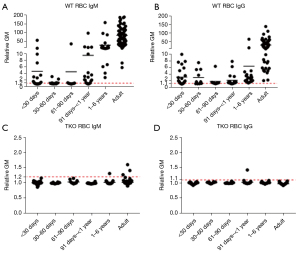
There continues to be concern for the potential of cross-species transmission of potentially infectious microorganisms, but expert opinion is that most post-transplant infections are likely to be the same nosocomial infections as those seen after allotransplantation (23). The presence of porcine endogenous retroviruses (PERV) in pig cells was a concern, but these can now be inactivated in the pig (24).
Community attitudes
The history, ethical considerations, and psychosocial impact surrounding xenotransplantation make it important to delineate community attitudes before clinical implementation. Early surveys distributed to pediatric medical providers and families of children with CHD indicate potentially high levels of acceptance (25-28). If the functional performance of the xenograft is proven inferior to that of an allograft, acceptance rates among stakeholders were lower (particularly for the use of a xenograft as a bridge to transplant). Unfortunately, these early surveys were limited by their broad scope and the limited background information provided to participants. A more focused follow-up study helped identify specific concerns (religious beliefs, animal ethics, stigma about how pigs are viewed, organ allocation logistics, and impact on quality of life) to target for improved acceptance by better education (29). Further studies are warranted to explore attitudes across providers and families from a broad scope of backgrounds to ensure widespread applicability of these findings.
Immune privilege of children
Pediatric application of xenotransplantation may offer immunologic advantages over the adult population. The case for this argument is made strong through understanding the outcomes of neonatal and infant cardiac allotransplantation in addition to the outcomes of a few key in vitro studies. Current ISHLT data indicate that neonates and infants with heart allografts experience less rejection, allograft vasculopathy, post-transplant lymphoproliferative disease, and need for re-transplant, but greater survival compared with all other age groups that receive a cardiac transplant. In fact, the half-life of a heart transplanted prior to one year of age has yet to be determined (2,30). Many argue that this superiority is driven by an immunologic advantage inherent to children less than one-year-old, i.e., inducibility. Research dating back to 1945 suggests that immune responses can be modified in young patients with early antigen exposure (30,31). However, such immunologic advantage remains ill-defined and difficult to target with therapies intended to augment it.
A second factor that must be taken into consideration is the fact that most neonates and infants undergo total thymectomy at the time of heart transplantation. Although this is not associated with many infectious complications, it certainly reduces the patient’s T cell response and may well contribute to the good results achieved after heart transplantation in this age group (31).
Cardiac allotransplantation across the ABO blood group barrier remains an excellent example of tolerance by the infant immune system. In 2000, West and colleagues theorized that, because human infants lack strong humoral responsiveness to stimulation by carbohydrate antigens and have low levels of antibodies to non-self A and B blood group antigens, they would accept grafts from ABO-incompatible donors (32). Not only were the transplants uniformly successful, with excellent survival and low rates of rejection, but follow-up studies indicated that patients who received an ABO-incompatible organ failed to develop specific antibodies to the donor blood group (33). They named this phenomenon “donor-specific B-cell tolerance”. We predict that infant humans will demonstrate a similarly blunted and accommodating humoral response to a xenograft (34).
To investigate this, our group performed early in vitro assessments of neonates and infants both pre- and post-cardiac surgery to determine their native reactivity to porcine antigens. No patients less than one-year-old had performed IgM or IgG antibodies to TKO pig red blood cells, regardless of whether they had undergone prior cardiac surgery (20). This was not the case in older children and adults, several of whom registered significant levels of antibodies to TKO pig antigens. Most intriguing, newborns who underwent a Norwood procedure, which requires implantation of an allograft patch for arch reconstruction and generous blood transfusion, did not express levels of anti-pig IgM or IgG that would result in humoral rejection (20). Further studies are necessary to delineate infant human cellular porcine immunity along with their humoral reactivity to nucleated cells from pigs.
Bridge to allotransplantation
New technology is most ideally applied in clinical settings where it addresses a large gap within current care paradigms. Xenotransplantation has the potential to target the problems associated with contemporary pediatric VAD support which weigh heavily on patients and the healthcare system. Mortality associated with pediatric VADs has improved but remains at 30% for all children placed on support as a bridge. Morbidity is also high, mostly centered on thrombosis and the complications of therapies intended to prevent it. Independent of underlying diagnosis, children on a VAD demonstrate a 30% stroke rate and 25% risk of life-threatening bleed during their time on support. Furthermore, 30% of children will develop a major infection during VAD support, in part because all devices have externalized components, but primarily due to ongoing nosocomial exposure (13). Patients with small body surface areas on MCS cannot be discharged from hospital. Patients successfully bridged require prolonged inpatient hospital stays, resulting in numerous infections and ultimately a heavy financial burden on the healthcare system.
Pretransplant MCS significantly increases hospital costs compared to those of recipients not on MCS (35), MCS adding almost $69,000 to the adjusted excess cost per case basis in post-operative CHD surgery (36). While the additional costs associated with infant cardiac xenotransplantation are hitherto unknown, the potential for early home discharge with outpatient follow-up until allotransplantation would almost certainly lower the costs compared to continued hospitalization of an infant supported by a currently available MCS (in addition, home care would be greatly beneficial from a psychological perspective for the patient and the family).
A fully implantable, biologic pump capable of delivering adequate biventricular cardiac output would revolutionize the current pediatric bridge-to-transplant paradigm. A xenograft’s natural endothelial lining would preclude the need for intensive anticoagulation. Our current standard of care in animal experiments of xenotransplantation is simply to give recipients only 40 mg of aspirin on alternate days. The xenograft’s lack of externalized hardware would eliminate the risk of infection inherent to exposed drivelines and cannulas. This may be a neutral benefit when accounting for the risks of required immunosuppression.
However, the graft’s ability to adjust to physiologic demands could allow for safe discharge to home during the bridge period. This would help reduce the length and cost of prolonged inpatient hospitalization along with the added benefit of diminishing exposure to nosocomial infections. As experience with the technology improves, the transplant community will likely support efforts to discharge xenotransplant recipients to home while retaining a stable United Network for Organ Sharing listing status.
There are, however, remaining barriers to the clinical application of cardiac xenotransplantation in infants. To date, no research group has demonstrated consistent six-month survival of an orthotopically-placed cardiac xenograft in an infant NHP. Nevertheless, all of the evidence to date indicates that the development of an immune response to a TKO pig heart will not result in the production of antibodies that cross-react with human leukocyte antigens (HLA) and so will not prevent successful subsequent allotransplantation (37).
As a research group, we are actively evaluating orthotopic cardiac transplants from TKO pigs into juvenile baboons (4–6 kg in weight). Our goal is to demonstrate consistent 4–6 months survival of life-supporting cardiac xenografts with no evidence of cross-reactivity of anti-pig antibodies with human antigens that would preclude subsequent allotransplantation. To ensure clinical feasibility, at 4–6 months we will excise the pig heart and replace it with a baboon allograft, which we would monitor for a further 2–4 months. If these milestones are achieved, we believe a clinical trial in infants failing traditional single ventricle palliation is warranted.
There is clearly a risk in this approach because, in the event of graft failure, there is likely to be no alternative therapy available. However, without a xenograft, the risk of death on MCS is also high. We would not proceed to a clinical trial unless our laboratory studies in the pig-to-baboon model indicated that a clinical trial would have a realistic chance of success. As NHPs are more likely to have preformed antibodies to TKO pig cells whereas our in vitro data indicate that no human infants have anti-pig antibodies (Figure 1) (and our current immunosuppressive regimen successfully prevents the production of de novo antibodies) we believe that rejection is unlikely to be problematic.
Selection of patients
If preclinical animal studies confirm an acceptable safety profile for orthotopic cardiac xenotransplantation, the question remains: “What clinical setting is the most appropriate for initial application?” Seventy percent of pre- or post-first stage single ventricle patients who need a VAD die before a suitable human heart can be found. The children within this group who receive heart allotransplants undergo MCS for a median of 64 days (13,38). Most successful experiences with bridging first stage single ventricle patients to transplant with a VAD occur in a few specialized centers around the country. Other single center experiences report mortality rates as high as 100% (39).
If this low success rate is exceeded clinically, populations with lower risk VAD profiles could be offered xenotransplantation as an alternative bridge in a hazard-adjusted fashion: (I) failed second stage single ventricles; (II) failed third stage single ventricles; (III) complex failed biventricular CHD; (IV) dilated/restrictive cardiomyopathies. Contrary to adult applications, porcine hearts used in these settings would eventually be removed in favor of a human allograft, which may also promote community acceptance and clinical expansion.
We believe there are significant advantages of our proposal to employ a pig xenograft as a bridge to allotransplantation. No NHP has yet survived beyond 9 months after orthotopic transplantation of a pig heart, and therefore the prospects for destination therapy are presently limited. Bridging of infants for 4–6 months would be much more feasible. Bridging does not commit an infant to a lifetime dependent on a pig heart which, in view of our limited knowledge of the field at present, must be an advantage.
Destination therapy
Looking further into the future, successfully bridging pediatric patients to transplant across all diagnoses could open the opportunity for destination therapy. This has precedent in adult VADs, which began initially as a therapy offered only as a bridge but eventually became destination therapy once results were proven superior to medical therapy alone (40). Large gaps in the current care paradigm of CHD leave room for xenotransplantation to play a role, particularly in the care of single ventricle patients.
The current limits of three-stage palliation
The evolution and refinement of three-stage single ventricle palliation has saved the lives of many children. As those children progressed into adulthood, the medical community discovered that Fontan physiology is not a lifelong solution. Instead, it represents a stable state while patients await inevitable cardiac transplantation to prolong their lives past the third and fourth decades (41-44). Furthermore, we are learning more about the long-term consequences of elevated central venous pressure on end organ function, including but not limited to hepatic, renal, gastrointestinal, pulmonary, neurologic, and lymphatic systems (45-49). Because of this end organ dysfunction as well as the added technical complexity and immunologic impact of multiple prior cardiac operations, adult Fontan patients demonstrate greater post-transplant mortality compared to their biventricular counterparts (50,51). Such profound limitations warrant reconsideration of a well proven fact—single ventricle palliation is offered because of necessity, not superiority among available therapies.
Although bridging by a xenograft will necessitate a second operation to replace the pig graft with an allograft, this is arguably preferable to the multiple palliation procedures and eventual cardiac allotransplant required by many patients.
Cardiac transplantation is superior to palliation
In the 1980s, a few centers in the United States pursued primary cardiac transplantation as the treatment modality for hypoplastic left heart syndrome (HLHS). Simultaneously, the remaining centers in the United States offered three-stage single ventricle palliation. The long-term survival advantage favoring human primary transplant is clear for newborns with HLHS. At 15 years from index operation, 65% of patients with primary transplants are alive versus 40% of three-stage palliation patients (52,53). In addition to better survival, the negative sequelae of prolonged elevation in central venous pressures were avoided in the transplant population. If enough donor hearts were available, cardiac replacement would be the treatment of choice for HLHS. Cardiac xenografts have the potential to fill this need assuming they demonstrate acceptable longevity, associated morbidity, and rates of rejection.
Prenatal diagnosis
Over the past two decades, there have been significant improvements in fetal diagnosis. In the present era, there is an expectation of accurate and detailed diagnosis of complex CHD in fetuses referred to pediatric cardiologists. Diagnosis before birth would allow sufficient time for counseling of the parents about the potential availability of xenotransplantation among other treatment options. If they wished to explore xenotransplantation, the time before birth would allow prenatal clinical preparation.
The ability to accurately diagnose CHD in the fetus also provides the possibility of modifying the neonatal immune response to xenoantigens and thereby improving the outcome of cardiac xenotransplantation. In fact, this ability could potentially result in cardiac xenotransplantation utilized as destination therapy for a group of neonates that have been documented to have poor prognosis.
Conclusions
Xenotransplantation with gene-edited porcine hearts offers great promise. There is a specific population of vulnerable neonates and infants with CHD who could immediately benefit from its introduction. Our community, comprised of physicians, nurses, families, and patients, must investigate and overcome its current limitations. Once these are resolved, we must boldly push towards clinical application.
Acknowledgments
Funding: None.
Footnote
Provenance and Peer Review: This article was commissioned by the Guest Editors (Antonio F. Corno and Ali Dodge-Khatami) for the column “Pediatric Heart” published in Translational Pediatrics. The article has undergone external peer review.
Peer Review File: Available at https://tp.amegroups.com/article/view/10.21037/tp-22-664/prf
Conflicts of Interest: All authors have completed the ICMJE uniform disclosure form (available at https://tp.amegroups.com/article/view/10.21037/tp-22-664/coif). The column “Pediatric Heart” was commissioned by the editorial office without any funding or sponsorship. J.D.C. and D.C.C. received grants from eGenesis Biotechnology. D.K.C.C. received consulting fees from eGenesis Biotechnology. The authors have no other conflicts of interest to declare.
Ethical Statement: The authors are accountable for all aspects of the work in ensuring that questions related to the accuracy or integrity of any part of the work are appropriately investigated and resolved.
Open Access Statement: This is an Open Access article distributed in accordance with the Creative Commons Attribution-NonCommercial-NoDerivs 4.0 International License (CC BY-NC-ND 4.0), which permits the non-commercial replication and distribution of the article with the strict proviso that no changes or edits are made and the original work is properly cited (including links to both the formal publication through the relevant DOI and the license). See: https://creativecommons.org/licenses/by-nc-nd/4.0/.
References
- Kirk R, Butts RJ, Dipchand AI. The first successful pediatric heart transplant and results from the earliest era. Pediatr Transplant 2019;23:e13349. [Crossref] [PubMed]
- Rossano JW, Singh TP, Cherikh WS, et al. The International Thoracic Organ Transplant Registry of the International Society for Heart and Lung Transplantation: Twenty-second pediatric heart transplantation report - 2019; Focus theme: Donor and recipient size match. J Heart Lung Transplant 2019;38:1028-41. [Crossref] [PubMed]
- Denfield SW, Azeka E, Das B, et al. Pediatric cardiac waitlist mortality-Still too high. Pediatr Transplant 2020;24:e13671. [Crossref] [PubMed]
- Alcorn JB. Changes to OPTN Bylaws and Policies from actions at June Board of Directors Meeting. July 2014. Available online: http://optn.transplant.hrsa.gov
- Choudhry S, Wang Y, Denfield SW, et al. A Recipient Risk Prediction Tool for Short-term Mortality After Pediatric Heart Transplantation. Transplantation 2019;103:2434-9. [Crossref] [PubMed]
- Schweiger M, Everitt MD, Chen S, et al. Review of the discard and/or refusal rate of offered donor hearts to pediatric waitlisted candidates. Pediatr Transplant 2020;24:e13674. [Crossref] [PubMed]
- Kirk R, Dipchand AI, Davies RR, et al. ISHLT consensus statement on donor organ acceptability and management in pediatric heart transplantation. J Heart Lung Transplant 2020;39:331-41. [Crossref] [PubMed]
- Mowers KL, Simpson KE, Gazit AZ, et al. Moderate-severe primary graft dysfunction after pediatric heart transplantation. Pediatr Transplant 2019;23:e13340. [Crossref] [PubMed]
- Davies RR, Bano M, Butts RJ, et al. Donor organ turn-downs and outcomes after listing for pediatric heart transplant. J Heart Lung Transplant 2019;38:241-51. [Crossref] [PubMed]
- Godown J, Kirk R, Joong A, et al. Variability in donor selection among pediatric heart transplant providers: Results from an international survey. Pediatr Transplant 2019;23:e13417. [Crossref] [PubMed]
- Beeman A, Muthialu N. ABO-incompatible heart transplantation in children-a systematic review of current practice. Indian J Thorac Cardiovasc Surg 2020;36:190-3. [Crossref] [PubMed]
- Rajab TK, Jaggers J, Campbell DN. Donation after circulatory death determination pediatric heart transplantation and 10-year outcomes. J Heart Lung Transplant 2020;39:491-2. [Crossref] [PubMed]
- Morales DLS, Adachi I, Peng DM, et al. Fourth Annual Pediatric Interagency Registry for Mechanical Circulatory Support (Pedimacs) Report. Ann Thorac Surg 2020;110:1819-31. [Crossref] [PubMed]
- Hardy JD, Chavez CM, Kurrus FD, et al. Heart transplantation in man. Developmental studies and report of a case. JAMA 1964;188:1132-40.
- Bailey LL, Nehlsen-Cannarella SL, Concepcion W, et al. Baboon-to-human cardiac xenotransplantation in a neonate. JAMA 1985;254:3321-9.
- Cozzi E, White DJ. The generation of transgenic pigs as potential organ donors for humans. Nat Med 1995;1:964-6. [Crossref] [PubMed]
- Cong L, Ran FA, Cox D, et al. Multiplex genome engineering using CRISPR/Cas systems. Science 2013;339:819-23. [Crossref] [PubMed]
- Mali P, Yang L, Esvelt KM, et al. RNA-guided human genome engineering via Cas9. Science 2013;339:823-6. [Crossref] [PubMed]
- Estrada JL, Martens G, Li P, et al. Evaluation of human and non-human primate antibody binding to pig cells lacking GGTA1/CMAH/β4GalNT2 genes. Xenotransplantation 2015;22:194-202. [Crossref] [PubMed]
- Li Q, Hara H, Banks CA, et al. Anti-Pig Antibody in Infants: Can a Genetically Engineered Pig Heart Bridge to Allotransplantation? Ann Thorac Surg 2020;109:1268-73. [Crossref] [PubMed]
- Cooper DKC, Hara H, Iwase H, et al. Justification of specific genetic modifications in pigs for clinical organ xenotransplantation. Xenotransplantation 2019;26:e12516. [Crossref] [PubMed]
- Griffith BP, Goerlich CE, Singh AK, et al. Genetically Modified Porcine-to-Human Cardiac Xenotransplantation. N Engl J Med 2022;387:35-44. [Crossref] [PubMed]
- Fishman JA. Prevention of infection in xenotransplantation: Designated pathogen-free swine in the safety equation. Xenotransplantation 2020;27:e12595. [Crossref] [PubMed]
- Niu D, Wei HJ, Lin L, et al. Inactivation of porcine endogenous retrovirus in pigs using CRISPR-Cas9. Science 2017;357:1303-7. [Crossref] [PubMed]
- Padilla LA, Hurst DJ, Jang K, et al. Racial differences in attitudes to clinical pig organ Xenotransplantation. Xenotransplantation 2021;28:e12656. [Crossref] [PubMed]
- Padilla LA, Rhodes L, Sorabella RA, et al. Attitudes toward xenotransplantation: A survey of parents and pediatric cardiac providers. Pediatr Transplant 2021;25:e13851. [Crossref] [PubMed]
- Padilla LA, Sorabella RA, Carlo WF, et al. Attitudes to Cardiac Xenotransplantation by Pediatric Heart Surgeons and Physicians. World J Pediatr Congenit Heart Surg 2020;11:426-30. [Crossref] [PubMed]
- Hurst DJ, Padilla LA, Cooper DKC, et al. Factors influencing attitudes toward xenotransplantation clinical trials: A report of focus group studies. Xenotransplantation 2021;28:e12684. [Crossref] [PubMed]
- Chinnock RE, Bailey LL. Heart transplantation for congenital heart disease in the first year of life. Curr Cardiol Rev 2011;7:72-84. [Crossref] [PubMed]
- Owen RD. IMMUNOGENETIC CONSEQUENCES OF VASCULAR ANASTOMOSES BETWEEN BOVINE TWINS. Science 1945;102:400-1. [Crossref] [PubMed]
- Bikhet M, Morsi M, Hara H, et al. The immune system in infants: Relevance to xenotransplantation. Pediatr Transplant 2020;24:e13795. [Crossref] [PubMed]
- West LJ, Pollock-Barziv SM, Dipchand AI, et al. ABO-incompatible heart transplantation in infants. N Engl J Med 2001;344:793-800. [Crossref] [PubMed]
- Fan X, Ang A, Pollock-Barziv SM, et al. Donor-specific B-cell tolerance after ABO-incompatible infant heart transplantation. Nat Med 2004;10:1227-33. [Crossref] [PubMed]
- Dons EM, Montoya C, Long CE, et al. T-cell-based immunosuppressive therapy inhibits the development of natural antibodies in infant baboons. Transplantation 2012;93:769-76. [Crossref] [PubMed]
- Ye XT, Parker A, Brink J, et al. Cost-effectiveness of the National Pediatric Heart Transplantation Program in Australia. J Thorac Cardiovasc Surg 2019;157:1158-1166.e2. [Crossref] [PubMed]
- Pasquali SK, He X, Jacobs ML, et al. Excess costs associated with complications and prolonged length of stay after congenital heart surgery. Ann Thorac Surg 2014;98:1660-6. [Crossref] [PubMed]
- Cooper DKC, Habibabady Z, Kinoshita K, et al. The respective relevance of sensitization to alloantigens and xenoantigens in pig organ xenotransplantation. Hum Immunol 2023;84:18-26. [Crossref] [PubMed]
- Bleiweis MS, Fudge JC, Peek GJ, et al. Ventricular assist device support in neonates and infants with a failing functionally univentricular circulation. JTCVS Tech 2022;13:194-204. [Crossref] [PubMed]
- Maeda K, Rosenthal DN, Reinhartz O. Ventricular Assist Devices for Neonates and Infants. Semin Thorac Cardiovasc Surg Pediatr Card Surg Annu 2018;21:9-14. [Crossref] [PubMed]
- Rose EA, Gelijns AC, Moskowitz AJ, et al. Long-term use of a left ventricular assist device for end-stage heart failure. N Engl J Med 2001;345:1435-43. [Crossref] [PubMed]
- Hernandez GA, Lemor A, Clark D, et al. Heart transplantation and in-hospital outcomes in adult congenital heart disease patients with Fontan: A decade nationwide analysis from 2004 to 2014. J Card Surg 2020;35:603-8. [Crossref] [PubMed]
- Kenny LA, DeRita F, Nassar M, et al. Transplantation in the single ventricle population. Ann Cardiothorac Surg 2018;7:152-9. [Crossref] [PubMed]
- Serfas JD, Thibault D, Andersen ND, et al. The Evolving Surgical Burden of Fontan Failure: An Analysis of The Society of Thoracic Surgeons Congenital Heart Surgery Database. Ann Thorac Surg 2021;112:179-87. [Crossref] [PubMed]
- Poh C, Hornung T, Celermajer DS, et al. Modes of late mortality in patients with a Fontan circulation. Heart 2020;106:1427-31. [Crossref] [PubMed]
- Dori Y, Smith CL. Lymphatic Disorders in Patients With Single Ventricle Heart Disease. Front Pediatr 2022;10:828107. [Crossref] [PubMed]
- John AS, Johnson JA, Khan M, et al. Clinical outcomes and improved survival in patients with protein-losing enteropathy after the Fontan operation. J Am Coll Cardiol 2014;64:54-62. [Crossref] [PubMed]
- Itkin M, Piccoli DA, Nadolski G, et al. Protein-Losing Enteropathy in Patients With Congenital Heart Disease. J Am Coll Cardiol 2017;69:2929-37. [Crossref] [PubMed]
- Zafar F, Lubert AM, Katz DA, et al. Long-Term Kidney Function After the Fontan Operation: JACC Review Topic of the Week. J Am Coll Cardiol 2020;76:334-41. [Crossref] [PubMed]
- Hilscher MB, Wells ML, Venkatesh SK, et al. Fontan-associated liver disease. Hepatology 2022;75:1300-21. [Crossref] [PubMed]
- Lamour JM, Kanter KR, Naftel DC, et al. The effect of age, diagnosis, and previous surgery in children and adults undergoing heart transplantation for congenital heart disease. J Am Coll Cardiol 2009;54:160-5. [Crossref] [PubMed]
- Backer CL, Russell HM, Pahl E, et al. Heart transplantation for the failing Fontan. Ann Thorac Surg 2013;96:1413-9. [Crossref] [PubMed]
- John MM, Razzouk AJ, Chinnock RE, et al. Primary Transplantation for Congenital Heart Disease in the Neonatal Period: Long-term Outcomes. Ann Thorac Surg 2019;108:1857-64. [Crossref] [PubMed]
- Stackhouse KA, McCrindle BW, Blackstone EH, et al. Surgical palliation or primary transplantation for aortic valve atresia. J Thorac Cardiovasc Surg 2020;159:1451-1461.e7. [Crossref] [PubMed]