Application value of metagenomics next-generation sequencing in the diagnosis of respiratory virus infection after congenital heart surgery
Highlight box
Key findings
• Using metagenomics next-generation sequencing (mNGS) on bronchoalveolar lavage fluid enhances the detection of respiratory virus infections (RVIs) following congenital heart surgery (CHS).
What is known, and what is new?
• mNGS offers a swift, efficient, and unbiased approach for obtaining microbial nucleic acid sequences. This technology has the potential to become a universal diagnostic tool for infectious diseases, particularly for pathogens undetectable by traditional diagnostic methods.
• mNGS can be used to diagnose RVIs following CHS.
What is the implication, and what should change now?
• mNGS allows for more efficient detection of coexisting viral infections after CHS. Based on these results, clinicians can tailor symptom management, adjust antiviral treatments, and modify infection control protocols, ultimately enhancing patient outcomes.
Introduction
Respiratory viral infections (RVIs) are frequent among infants and young children. The most common causative agent is the respiratory syncytial virus (RSV) (1). Remarkably, about 95% of children aged under 2 years contract RSV, making it a leading cause of hospitalization in this age group (1,2). Aside from RSV, other viruses such as rhinovirus, influenza, parainfluenza virus, human metapneumovirus, coronavirus, and bocavirus have been identified in patients presenting with respiratory symptoms (3). For children with congenital heart disease (CHD), RVIs can prolong hospital and intensive care unit stays, increase the duration of mechanical ventilation, delay elective cardiac surgeries, and in severe patients, lead to mortality (4-6).
Several methods exist for RVI detection, including virus culture, immunological techniques, and polymerase chain reaction (PCR). Virus culture is considered the “gold standard”, but is often impractical for routine clinical laboratory use because of its complexity, time-consuming nature, and demanding technical requirements. Immunological techniques offer simplicity but have limitations. For instance, immunoglobulin M antibodies typically do not appear until at least 6 days post-infection, and previous infections can sometimes lead to false-positive results. Moreover, the sensitivity and specificity of these techniques could benefit from enhancements. The PCR method, while both sensitive and specific, generally detects only one to three viruses in a single reaction, limiting its capacity (7,8). In the clinical management of CHD complicated by RVI, diagnostic clarity can vary widely across healthcare settings. Uncertain and delayed diagnoses often push clinicians toward empirical treatments, which might result in inappropriate drug use and therapeutic delays. Given these challenges, there is a pressing need for a diagnostic method that is accurate, swift, comprehensive, highly sensitive, and highly specific for RVI detection.
Bronchoalveolar lavage fluid (BALF) is a cornerstone diagnostic procedure for pulmonary diseases and is routinely used in pneumonia diagnosis. Traditional etiological detection techniques, such as culture, immunological assays, and PCR, have been the mainstay of clinical pneumonia diagnosis. However, these methods have limitations, including delayed results, limited pathogen detection scope, and a suboptimal rate of positive detections.
Metagenomics next-generation sequencing (mNGS) stands out as a groundbreaking technology that offers the rapid, efficient, and unbiased retrieval of microbial nucleic acid sequence data (9). Ever since its pioneering application in diagnosing Leptospirosis in 2014, mNGS has gained increasing appreciation for its diagnostic merits (10). With its hallmark features, including its high sensitivity, swift result turnover, unbiased detection, and culture independence, mNGS has solidified its position as an innovative and powerful tool for diagnosing infectious diseases, particularly when conventional diagnostics fall short (11).
The prowess of mNGS in identifying microbes in infectious diseases and severe pneumonia patients is evident; however, its specific role in detecting postoperative infections following congenital heart surgery (CHS) has not yet been fully elucidated. In this study, we undertook a retrospective analysis of clinical data to ascertain the diagnostic value of mNGS for RVIs post-CHS. We present this article in accordance with the STROBE reporting checklist (available at https://tp.amegroups.com/article/view/10.21037/tp-23-341/rc).
Methods
Ethical approval
The study was conducted in accordance with the Declaration of Helsinki (as revised in 2013). The study was approved by the ethics board of Fujian Children’s Hospital (No. 2022ETKLR12043) and informed consent was taken from all the patients’ parents or legal guardians.
Population and study criteria
The data of patients who underwent surgery for CHD and subsequently developed respiratory tract infections were retrospectively analyzed. These patients were admitted to the cardiac intensive care unit (CICU) of Fujian Children’s Hospital between July 2021 and December 2022. The clinical data for each patient were extracted from their electronic medical records, including their demographic details, specific type of CHD, anti-infective medication use, routine blood test results, inflammatory markers, duration of stay in the CICU, and eventual outcomes.
To be eligible for inclusion in this study, the patients had to meet the following inclusion criteria: (I) have been diagnosed with CHD and have undergone cardiac surgery; and (II) have symptoms of respiratory tract infection, including cough, wheezing, rhinorrhea, fever and, in more severe patients, shortness of breath and dyspnea (12). For the patients from whom BALF was collected by bedside bronchoscopy, if the cause of infection remained unidentified and empirical anti-infective treatment proved ineffective after 3 days, mNGS was suggested, and if their parents agreed to allow them to undergo mNGS, they were included in the mNGS group. Conversely, if mNGS was refused, often due to its higher cost, the patients were placed in the conventional microbiological test (CMT) group. The patients in the latter group underwent standard microbiological tests, including respiratory virus antigen detection, sputum culture, routine laboratory smear staining, real-time PCR, enzyme-linked immunospot assay, GeneXpert, and serum 1,3-β-D-glucan tests.
Patients were excluded from the study if they met any of the following exclusion criteria: (I) had symptoms of respiratory tract infection but had predominant symptoms that were more indicative of congestive heart failure, such as liver or lung congestion, peripheral edema, or reduced cardiac function; (II) had refused to undergo the bronchoscopy procedure to collect BALF; (III) had BALF specimens that did not meet the quality standards for mNGS, such as those with more than 99% human-derived sequences; (IV) had samples affected by leakage or contamination issues; and/or (V) had incomplete clinical or laboratory records.
The primary outcome of interest was the detection rate of respiratory viruses in the study participants diagnosed with viral pneumonia. Based on a literature review (13), the anticipated virus detection rate for the mNGS group was 61%, while that for the CMT group was 24%. Given a two-sided significance level of 0.05 and a power of 90%, the sample-size calculations were made using PASS 15 software. The results indicated that at least 33 participants would be needed for the mNGS group (N1 =33) and another 33 participants would be needed for the CMT group (N2 =33). However, to account for potential loss to follow-up and a refusal rate of 20%, it was decided that a minimum of 42 participants would be recruited for each group, resulting in a total of at least 84 participants for the study.
General information
We documented the demographic details of the patients, including gender, age, and weight. We also noted the specific type of CHD they were diagnosed with, their usage pattern of anti-infective medications, and their routine blood test results. We also took into account various inflammatory indicators, such as white blood cell (WBC) count, C-reactive protein (CRP), procalcitonin (PCT), and pro-brain natriuretic peptide (pro-BNP), which were measured within 24 hours before the specimen examination.
The primary outcomes included a comparison of the virus detection rates from the two distinct methods, the rate of detection of mixed viral infections using both methods, and the utilization rate of antiviral medications. The secondary outcomes were based on metrics and included the rate of improvement in respiratory symptoms, the duration of postoperative mechanical ventilation, the period of stay in the CICU, and the cumulative hospitalization duration.
Sample processing and routine microbiological testing
After obtaining written informed consent from the patients or their legal guardians, the attending physician carried out a bedside bronchoscopy. The procedure, which was executed using a fiberoptic bronchoscope, adhered to established standards. Patients’ heart rate, blood pressure, and pulse oxygen saturation were continuously monitored throughout the procedure. After the treatment, 3–5 mL of BALF was collected in a sterile sputum container, which was then dispatched to the microbiology laboratory for further tests. Both mNGS and CMT tests were conducted, including microbial isolation and culture, smear microscopy, antibody and antigen detection, and PCR. It was critical that the BALF specimen was promptly relayed to the laboratory and processed without delay, ideally within an hour of collection. If immediate processing was not feasible, the specimen was stored at a temperature of −70 °C or lower. When transported to the laboratory, freezing conditions were maintained, and it was ensured that the duration did not exceed 24 hours. After the exclusion of any background pathogens, a positive result on any of the standard etiological tests, in congruence with clinical characteristics, was considered a confirmed positive outcome.
mNGS methods and bioinformatics analysis
The 3–5-mL BALF sample was processed following standardized procedures. A vortex mixer’s horizontal platform held a 1.5-mL microcentrifuge tube containing 0.5 mL of the BALF sample and 1 g of 0.5-mm glass beads. This solution was rigorously agitated at 3,000 rpm for roughly half an hour. After agitation, 0.3 mL of the sample was separated into a new 1.5-mL microcentrifuge tube, and deoxyribonucleic acid (DNA) was extracted using a TIANamp Micro DNA Kit (DP316, Tiangen Biotech, Beijing, China) in accordance with the manufacturer’s instructions. DNA libraries were subsequently established through DNA fragmentation, end repair, adapter ligation, and PCR enhancement. Agilent 2100 (Agilent, Santa Clara, CA, USA) was used for DNA library quality control. The qualified libraries were sequenced by the BGISEQ-50 platform (14).
Using high-throughput sequencing technology, the nucleic acid sequences of the microorganisms in the samples were analyzed. These sequences were identified by comparing them against existing microorganism nucleic acid sequences in specialized databases. The detection procedure covered pretreatment, nucleic acid extraction, library formulation [preparation of the DNA libraries, including end repair, adaptor ligation, PCR enrichment, purification, clone generation, and preparation of the ribonucleic acid (RNA) libraries, including ribosome removal, reverse transcription and hybridization, fragmentation, first/second strand synthesis, end repair, adaptor ligation, PCR enrichment, purification, and cloning], sequencing, bioinformatics evaluation, and result interpretation. The MGISEQ-2000 quality control was performed using the Agilent 2100 Bioanalyzer system, and the qualified libraries were sequenced on the BGISEQ 50 platform. Quality mandates included a sequencing data volume of ≥20 M, a sequencing read length >50 bp, over 90% dehumanized sequences, and a pathogen database that comprised >10,000 species. This particular database integrated genomes of diverse entities, such as archaea, bacteria, fungi, protozoa, viruses, and parasites, all sourced from the NCBI Genome Database (15). The number of unique alignment reads was calculated and normalized to obtain the number of reads strictly localized to the pathogen species and those strictly localized to the pathogen genus. Figure 1 shows the mNGS workflow.
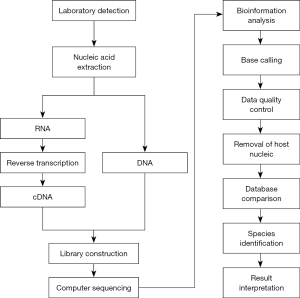
The following criteria were established for a positive mNGS result: (I) bacteria (excluding mycobacteria): a minimum of 50 reads aligned directly to the pathogen species, and a read number surpassing any other microorganism, or a read backed by CMT findings; (II) fungi, mycoplasma, chlamydia, and viruses: a read linked directly to a pathogen species that was at least five times higher than any other fungi or was confirmed by CMT outcomes; (III) mycobacterium tuberculosis: at least one distinct sequence matching the reference genome on either the genus or species level. This was due to the complexities associated with nucleic acid extraction and a slim chance of environmental contamination; and/or (IV) non-tuberculous mycobacterium (NTM): the relative bacterial abundance ranked within the top 10 of the bacterial list, due to the frequent occurrence of environmental contamination (16).
Statistical analysis
SPSS 26.0 software (SPSS Inc., Chicago, IL, USA) was used for the statistical analysis of the data collected in this research. Quantitative data with a normal distribution are presented as the mean ± standard deviation, and the t-test was used for comparisons. Quantitative data with a non-normal distribution are presented as the median and interquartile range (IQR), and the Mann-Whitney U-test was used for comparisons. Enumerative data are presented as either the value or percentage (%), and the χ2 test or Fisher’s exact test was used for group comparisons. A two-sided P value <0.05 was considered statistically significant.
Results
Demographic characteristics
We performed a retrospective analysis of 206 CHD patients who also had respiratory tract infections. Upon applying the exclusion criteria, 74 patients were excluded from the study for the following reasons: 40 declined the bronchoscopy procedure, 24 were not subjected to the necessary etiological tests, seven lacked a comprehensive clinical history, one did not pass the mNGS quality check, and two had contaminated BALF samples. After the exclusion process, 132 participants remained, of whom 62 were categorized to the mNGS group and 70 were categorized to the CMT group.
Table 1 presents the clinical characteristics of the patients in both groups, including gender, age, weight, WBC, CRP, PCT, pro-BNP taken within 24 hours preceding specimen collection, and the specific type of CHD. The statistical analysis revealed no statistically significant differences in these fundamental clinical characteristics between the mNGS and CMT groups (P>0.05). It should be noted that all the patients included in the study underwent diagnosis or treatment that involved bronchoscopy and BALF examinations.
Table 1
Characteristics | mNGS (n=62) | CMT (n=70) | P value |
---|---|---|---|
Gender (male/female) | 36/26 | 44/26 | 0.574 |
Age (months) | 3.56±0.62 | 2.88±0.46 | 0.372 |
Weight (kg) | 4.79±0.25 | 4.32±0.23 | 0.174 |
WBC (109/L) | 12.61±0.65 | 11.55±0.55 | 0.214 |
CRP (mg/L) | 22.30±3.46 | 17.67±3.25 | 0.331 |
PCT (ng/L) | 7.56±2.38 | 4.97±1.88 | 0.402 |
pro-BNP (pg/mL) | 8,427.35±1,400.24 | 6,450.56±1,211.36 | 0.290 |
CPB time (min) | 152.13±11.41 | 133.53±12.69 | 0.282 |
Aortic cross-clamp time (min) | 72.94±6.83 | 61.32±4.85 | 0.209 |
Types of CHD | >0.999 | ||
VSD | 31 (50.00) | 33 (47.14) | |
ASD | 4 (6.45) | 6 (8.57) | |
PDA | 11 (17.74) | 12 (17.14) | |
TOF | 2 (3.23) | 2 (2.86) | |
TGA | 1 (1.61) | 2 (2.86) | |
PS | 5 (8.06) | 7 (10.0) | |
DAA | 1 (1.61) | 2 (2.86) | |
PMV | 1 (1.61) | 1 (1.43) | |
MI | 3 (4.84) | 2 (2.86) | |
TAPVC | 3 (4.84) | 3 (4.29) |
Data are reported as the number and percentage or mean ± standard deviation. mNGS, metagenomic next-generation sequencing; CMT, conventional microbiological test; WBC, white blood cell; CRP, C-reactive protein; PCT, procalcitonin; pro-BNP, pro-brain natriuretic peptide; CPB, cardiopulmonary bypass; CHD, congenital heart disease; VSD, ventricular septal defect; ASD, atrial septal defect; PDA, patent ductus arteriosus; TOF, tetralogy of Fallot; TGA, transposition of the great arteries; PS, pulmonary stenosis; DAA, double aortic arch; PMV, prolapse of mitral valve; MI, mitral insufficiency; TAPVC, total anomalous pulmonary venous connection.
Comparison of mNGS and conventional detection methods
Of the 62 patients in the mNGS group, 49 tested positive and 13 tested negative. Among those who tested positive, 12 different viruses were identified in 35 patients, of which the three most frequently detected viruses were human herpes virus 5, human herpes virus 7, and rhinovirus A (see Table 2). Notably, of these patients, seven patients had one type of viral infection, while the remaining 28 had mixed virus infections (for further details, see Figure 2).
Table 2
Virus | Example number |
---|---|
Human herpesvirus 5 | 20 |
Human herpesvirus 7 | 8 |
Rhinovirus A | 6 |
Human adenovirus C | 4 |
Human herpes virus 4 | 3 |
Rhinovirus C | 3 |
Human adenovirus B | 3 |
Human bocavirus 1 | 2 |
Human respiratory syncytial virus A | 2 |
Human parainfluenza virus 3 | 1 |
Human corona virus | 1 |
Enterovirus | 1 |
mNGS, metagenomic next-generation sequencing.
Of the 70 patients in the CMT group, 25 tested positive and 45 tested negative. Among the positive patients, the following four distinct viruses were found in 12 patients: RSV (five patients), parainfluenza virus (three patients), herpes simplex virus (three patients), and cytomegalovirus (one patient). Notably, of these patients, 10 had only one type of viral infection, while the other two had mixed infections comprising RSV combined with Staphylococcus aureus.
A statistical analysis revealed significant differences between the two groups in terms of the virus detection rates, virus mixed-infection rates, and overall pathogen detection rates (P<0.001) (see Table 3).
Table 3
Variables | Detection situation | mNGS, n (%) | CMT, n (%) | P value |
---|---|---|---|---|
Total pathogen detection rate | Positive | 49 (79.03) | 25 (35.71) | <0.001 |
Negative | 13 (20.97) | 45 (64.29) | ||
Virus detection rate | Positive | 35 (56.45) | 12 (17.14) | |
Negative | 27 (43.55) | 58 (82.86) | ||
Virus mixed-infection detection rate | Single | 7 (20.00) | 10 (83.33) | |
Mixed | 28 (80.00) | 2 (16.67) |
mNGS, metagenomic next-generation sequencing; CMT, conventional microbiological test.
Treatment and prognosis
Based on the pathogen detection results, we modified the treatment approaches using the following guidelines: (I) antiviral treatment was tailored to both the virus detection findings from mNGS and the clinical manifestations; for patients with human herpes virus infections, ganciclovir was administered, while for those with RSV infections, aerosolized ribavirin therapy was administered; (II) symptomatic and supportive care was based on mNGS virus detection outcomes: (i) recombinant human interferon α-2b, ranging from 6–10 µg, was diluted in a 0.9% saline solution. The dose was generally 1–10 mL according to the symptoms of each patient for a duration of five days; (ii) immunoglobulin, at a dose of 200–400 mg/kg/day, was continuously administered for 5 days to bolster the immune response; (iii) adjunctive aerosolized inhalation treatments, including ambroxol hydrochloride and other antispasmodic and anti-asthmatic medications, were given to decrease airway resistance; and (III) the anti-infective strategy was revised in line with each patient’s clinical presentation and the detected pathogens: (i) if new infectious agents were identified, the antibiotic regimen was escalated or altered; and (ii) in the absence of new infections and with stable disease presentation, antibiotics was reduced or deescalated. Antiviral therapy was only initiated in our study when the existing anti-infection approach was ineffective and when the symptoms aligned with those of pneumonia induced by the identified virus.
In the mNGS group, 12 (19.35%) patients received antiviral therapy, 38 (61.29%) had anti-infective regimen modifications, and ultimately, 46 (74.19%) showed improvements in their respiratory tract infection symptoms. Conversely, in the CMT group, antiviral therapy was adjusted for 3 (4.29%) patients, the anti-infective regimen was modified for 20 (28.57%) patients, and ultimately, 31 (44.29%) reported alleviated respiratory tract infection symptoms. The differences among the above three were statistically significant.
The mNGS group received mechanical ventilation for a shorter duration than the CMT group (3.37±0.37 vs. 5.67±1.00 days, P=0.036). Similarly, their stay in the CICU was shorter (5.58±0.48 vs. 7.2±0.41 days, P=0.012). The mNGS group also had a shorter overall hospital stay than the CMT group, but the difference was not statistically significant. Regrettably, one patient in the mNGS group and two patients in the CMT group experienced deteriorating symptoms, progressing to severe pneumonia, which resulted in their demise (see Table 4).
Table 4
Variables | mNGS | CMT | P value |
---|---|---|---|
Antiviral therapy | 12 (19.35) | 3 (4.29) | 0.014 |
Anti-infective regimen adjustment | 38 (61.29) | 20 (28.57) | <0.001 |
Respiratory tract infection symptoms improved | 46 (74.19) | 31 (44.29) | 0.001 |
Postoperative invasive ventilation time (days) | 3.37±0.37 | 5.67±1.00 | 0.036 |
Length of CICU stay (days) | 5.58±0.48 | 7.2±0.41 | 0.012 |
Total length of hospitalization (days) | 12.83±0.78 | 15.12±1.07 | 0.086 |
Number of in-hospital deaths | 1 (1.61) | 2 (2.86) | >0.999 |
Data are reported as the number and percentage or mean ± standard deviation. mNGS, metagenomic next-generation sequencing; CMT, conventional microbiological test; CICU, cardiac intensive care unit.
Discussion
Patients with CHD exhibit pathophysiological risk factors that may precipitate more severe clinical outcomes during RVI. CHD patients experience altered pulmonary mechanics, diminished lung compliance, and heightened pulmonary vascular resistance. Consequently, these patients are at an increased risk of RVI, especially post open-heart surgery (17). Further, children with left-to-right shunt anomalies can experience augmented pulmonary blood flow, inducing pulmonary edema, and a reduced functional residual capacity. Such mechanical alterations can escalate atelectasis and ventilation-perfusion imbalances, culminating in hypoxia (18).
Cardiac surgical procedures, including cardiopulmonary bypass, might exacerbate pulmonary hypertension initiated by viral infections and revive viral or inflammatory reactions, amplifying the severity of pneumonia and bronchial blockage. Other factors that might exacerbate post-surgery progression include a heightened likelihood of secondary bacterial pneumonia due to ongoing airway and alveolar inflammation and an inferior nutritional and immunological state (19).
We conducted an etiological analysis of BALF using mNGS, and found a high prevalence of respiratory virus infections (56.45%) and mixed viral infections (80%) post-CHS. The most commonly detected respiratory viruses in our study were human herpesvirus 5, human herpesvirus 7, rhinovirus A, and human adenovirus C. Conversely, Yang et al. applied mNGS to BALF samples from children with pneumonia and reported a viral detection rate of 61% and a viral mixed-infection rate of 69.34%. Their primary viral pathogens were human herpes viruses and human adenoviruses, particularly human cytomegalovirus and human adenovirus 7 (13). These findings align with our detection results. The higher frequency of mixed viral infections in our study might be attributed to the inclusion of patients displaying pronounced symptoms and thus having a heightened risk of concurrent infections of other pathogens.
In our study, the RVIs of the mNGS group were predominantly of a mixed-infection nature, which is consistent with the findings of Cilla et al., who reported the frequent co-occurrence of Streptococcus pneumoniae and assorted respiratory viruses in pediatric patients with community-acquired pneumonia. Notably, up to 45% of these pediatric patients displayed evidence of virus-bacterial mixed infections (20). In regions with developing healthcare infrastructure, both viruses and bacteria are often identified directly from lung aspirates of pneumonia patients. In Forgie’s study, 45 of 74 children were infected with pneumococcal community-acquired pneumonia, and viral culture or serologic testing provided evidence of RVI in 15 patients (21). Further, Johnson et al. conducted virological assessments on 122 pediatric patients with community-acquired pneumonia, and reported that 61 (50%) had viral infections. Among whom, 10 (16%) also showed bacterial presence in their blood cultures, of which Staphylococcus aureus was the most prevalent. This correlates with findings for our CMT group in which co-infections of RSV and Staphylococcus aureus were identified (22).
In our research, a clear correlation was observed between the presence of RVI and postoperative outcomes following CHS, which supports the findings of previous studies. Delgado-Corcoran et al. examined the effects of human rhinovirus infection in cardiac surgery patients and found that post-CHS patients, in whom rhinovirus was detected, experienced extended periods on mechanical ventilation, encountered more extubation challenges, required bronchodilators more frequently, and endured longer hospital stays than asymptomatic patients post-surgery (23). Similarly, Altman et al. examined the consequences of RSV infections in pediatric patients with CHD and found that such infections precipitated heightened morbidity, elevated mortality rates, and increased healthcare-related expenditures (24). However, Silva et al.’s examination of the prevalence of viral respiratory tract infections in pediatric CHS patients offered a different perspective. Of 48 participants, 16 (33.3%) were diagnosed with RVI before surgery, while four (8.3%) developed new infections postoperatively. Notably, there was no discernible difference in the duration of mechanical ventilation or in extubation failures between these two subsets of patients. This might be due to the fact that the majority of the subjects in the study were asymptomatic. The reliance on PCR for virus detection, combined with a prolonged detection window, meant that the treatment modalities were not promptly adjusted for patients where the virus was identified (17).
The diverse clinical presentations of RVI stem from the myriad of viruses in existence, many of which possess a high propensity for mutation. As a diagnostic method, mNGS has a distinct advantage over conventional viral etiological tests. Its capability to simultaneously identify thousands of viruses in a single test, without being contingent on any prior clinical hypothesis, makes its detection scope vast, quick, and impartial (25). Moreover, mNGS offers unparalleled benefits in terms of multi-pathogenic or intricate infections. It stands out due to its elevated detection rates for viruses and unconventional pathogens. The capacity of the technology to concurrently recognize multiple pathogens not only streamlines the diagnostic process but also expedites the turnaround time, aiding in timely therapeutic interventions (26). Zheng et al. showed the efficacy of mNGS in discerning the causative agents behind severe pneumonia post-CHS in young children and infants. The method elevates pathogen detection sensitivity, facilitates the pinpointing of suitable antibacterial treatments, and refines the precision of disease diagnosis and outcome prediction (27).
Within the purview of our research, patients identified with respiratory viruses were accorded symptomatic, supportive, and antiviral treatments. For example, after an initially unsuccessful empirical anti-infective regimen, Stenotrophomonas maltophilia was detected in one patient from the mNGS cohort. However, with the subsequent administration of trimethoprim-sulfamethoxazole, the pulmonary infection was decisively managed (28). Crucially, our findings indicate a marked enhancement in the rate of respiratory symptom alleviation in the mNGS group compared to the CMT group. Further, metrics such as the duration of mechanical ventilation, overall hospital stay, and the length of stay within the CICU were notably shorter in the mNGS group than the CMT group. Such outcomes are suggestive of a better prognosis and an overall improved patient recovery trajectory.
The current high testing cost is a significant barrier limiting the widespread use of mNGS in routine clinical settings. While the price of sequencing has experienced a considerable reduction since 2014, the average cost for an mNGS test ($500) still surpasses that of a single conventional etiological test ($300). That said, given its capability to identify all potential pathogens in one test, in the long term, mNGS might be more economical than a series of standard pathogen screenings (29). The technical intricacies linked with mNGS also act as a deterrent for its broad-scale adoption. There is an urgent need for more prospective clinical research and financial assessments that focus on evaluating the cost-efficiency of mNGS in enhancing patient health outcomes.
Our study had several limitations. First, the participants were recruited from a single medical center, and the number of study participants was relatively small. Second, this was a retrospective study, and certain biases, such as selectivity bias, could affect the accuracy of our findings. Third, due to sample-size constraints, we could not simultaneously compare mNGS with some other laboratory assays, such as panels and viral cultures. Finally, the participants enrolled in the study had all been admitted to the CICU and thus were all critically ill. Therefore, our findings might not apply to patients with mild disease.
Conclusions
Using mNGS for BALF enhances the detection of RVIs and coexisting viral infections post-CHS. This facilitates more precise treatment strategies and could potentially lead to improved patient outcomes.
Acknowledgments
We highly acknowledge all the staff from our unit who participated in this study.
Funding: None.
Footnote
Reporting Checklist: The authors have completed the STROBE reporting checklist. Available at https://tp.amegroups.com/article/view/10.21037/tp-23-341/rc
Data Sharing Statement: Available at https://tp.amegroups.com/article/view/10.21037/tp-23-341/dss
Peer Review File: Available at https://tp.amegroups.com/article/view/10.21037/tp-23-341/prf
Conflicts of Interest: All authors have completed the ICMJE uniform disclosure form (available at https://tp.amegroups.com/article/view/10.21037/tp-23-341/coif). The authors have no conflicts of interest to declare.
Ethical Statement: The authors are accountable for all aspects of the work in ensuring that questions related to the accuracy or integrity of any part of the work are appropriately investigated and resolved. The study was conducted in accordance with the Declaration of Helsinki (as revised in 2013). The study was approved by the ethics board of Fujian Children’s Hospital (No. 2022ETKLR12043) and informed consent was taken from all the patients’ parents or legal guardians.
Open Access Statement: This is an Open Access article distributed in accordance with the Creative Commons Attribution-NonCommercial-NoDerivs 4.0 International License (CC BY-NC-ND 4.0), which permits the non-commercial replication and distribution of the article with the strict proviso that no changes or edits are made and the original work is properly cited (including links to both the formal publication through the relevant DOI and the license). See: https://creativecommons.org/licenses/by-nc-nd/4.0/.
References
- Falkenstein-Hagander K, Månsson AS, Redmo J, et al. Viral aetiology and clinical outcomes in hospitalised infants presenting with respiratory distress. Acta Paediatr 2014;103:625-9. [Crossref] [PubMed]
- Granbom E, Fernlund E, Sunnegårdh J, et al. Respiratory Tract Infection and Risk of Hospitalization in Children with Congenital Heart Defects During Season and Off-Season: A Swedish National Study. Pediatr Cardiol 2016;37:1098-105. [Crossref] [PubMed]
- Petrarca L, Nenna R, Frassanito A, et al. Human bocavirus in children hospitalized for acute respiratory tract infection in Rome. World J Pediatr 2020;16:293-8. [Crossref] [PubMed]
- Medrano C, Garcia-Guereta L, Grueso J, et al. Respiratory infection in congenital cardiac disease. Hospitalizations in young children in Spain during 2004 and 2005: the CIVIC Epidemiologic Study. Cardiol Young 2007;17:360-71. [Crossref] [PubMed]
- Li X, Wang X, Li S, et al. Viral Respiratory Infection, a Risk in Pediatric Cardiac Surgery: A Propensity-Matched Analysis. Pediatr Crit Care Med 2020;21:e431-40. [Crossref] [PubMed]
- Diller GP, Enders D, Lammers AE, et al. Mortality and morbidity in patients with congenital heart disease hospitalised for viral pneumonia. Heart 2021;107:1069-76. [Crossref] [PubMed]
- Chartrand C, Tremblay N, Renaud C, et al. Diagnostic Accuracy of Rapid Antigen Detection Tests for Respiratory Syncytial Virus Infection: Systematic Review and Meta-analysis. J Clin Microbiol 2015;53:3738-49. [Crossref] [PubMed]
- Labelle AJ, Arnold H, Reichley RM, et al. A comparison of culture-positive and culture-negative health-care-associated pneumonia. Chest 2010;137:1130-7. [Crossref] [PubMed]
- Gu W, Miller S, Chiu CY. Clinical Metagenomic Next-Generation Sequencing for Pathogen Detection. Annu Rev Pathol 2019;14:319-38. [Crossref] [PubMed]
- Wilson MR, Naccache SN, Samayoa E, et al. Actionable diagnosis of neuroleptospirosis by next-generation sequencing. N Engl J Med 2014;370:2408-17. [Crossref] [PubMed]
- Duan J, Gao J, Liu Q, et al. Characteristics and Prognostic Factors of Non-HIV Immunocompromised Patients With Pneumocystis Pneumonia Diagnosed by Metagenomics Next-Generation Sequencing. Front Med (Lausanne) 2022;9:812698. [Crossref] [PubMed]
- Joshi M, Tulloh RM. Respiratory virus prophylaxis in congenital heart disease. Future Cardiol 2018;14:417-25. [Crossref] [PubMed]
- Yang A, Chen C, Hu Y, et al. Application of Metagenomic Next-Generation Sequencing (mNGS) Using Bronchoalveolar Lavage Fluid (BALF) in Diagnosing Pneumonia of Children. Microbiol Spectr 2022;10:e0148822. [Crossref] [PubMed]
- Fang C, Zhong H, Lin Y, et al. Assessment of the cPAS-based BGISEQ-500 platform for metagenomic sequencing. Gigascience 2018;7:1-8. [Crossref] [PubMed]
- Benson DA, Cavanaugh M, Clark K, et al. GenBank. Nucleic Acids Res 2013;41:D36-42. [Crossref] [PubMed]
- Miao Q, Ma Y, Wang Q, et al. Microbiological Diagnostic Performance of Metagenomic Next-generation Sequencing When Applied to Clinical Practice. Clin Infect Dis 2018;67:S231-40. [Crossref] [PubMed]
- Silva THD, Pinho JRR, Silva TJD Junior, et al. Epidemiology of viral respiratory infections in children undergoing heart surgery. Prog Pediatr Cardiol 2019;52:22-5. [Crossref] [PubMed]
- Geskey JM, Cyran SE. Managing the morbidity associated with respiratory viral infections in children with congenital heart disease. Int J Pediatr 2012;2012:646780. [Crossref] [PubMed]
- Khongphatthanayothin A, Wong PC, Samara Y, et al. Impact of respiratory syncytial virus infection on surgery for congenital heart disease: postoperative course and outcome. Crit Care Med 1999;27:1974-81. [Crossref] [PubMed]
- Cilla G, Oñate E, Perez-Yarza EG, et al. Viruses in community-acquired pneumonia in children aged less than 3 years old: High rate of viral coinfection. J Med Virol 2008;80:1843-9. [Crossref] [PubMed]
- Forgie IM, O'Neill KP, Lloyd-Evans N, et al. Etiology of acute lower respiratory tract infections in Gambian children: I. Acute lower respiratory tract infections in infants presenting at the hospital. Pediatr Infect Dis J 1991;10:33-41. [Crossref] [PubMed]
- Johnson AW, Osinusi K, Aderele WI, et al. Etiologic agents and outcome determinants of community-acquired pneumonia in urban children: a hospital-based study. J Natl Med Assoc 2008;100:370-85. [Crossref] [PubMed]
- Delgado-Corcoran C, Witte MK, Ampofo K, et al. The impact of human rhinovirus infection in pediatric patients undergoing heart surgery. Pediatr Cardiol 2014;35:1387-94. [Crossref] [PubMed]
- Altman CA, Englund JA, Demmler G, et al. Respiratory syncytial virus in patients with congenital heart disease: a contemporary look at epidemiology and success of preoperative screening. Pediatr Cardiol 2000;21:433-8. [Crossref] [PubMed]
- Lewandowska DW, Schreiber PW, Schuurmans MM, et al. Metagenomic sequencing complements routine diagnostics in identifying viral pathogens in lung transplant recipients with unknown etiology of respiratory infection. PLoS One 2017;12:e0177340. [Crossref] [PubMed]
- Li N, Ma X, Zhou J, et al. Clinical application of metagenomic next-generation sequencing technology in the diagnosis and treatment of pulmonary infection pathogens: A prospective single-center study of 138 patients. J Clin Lab Anal 2022;36:e24498. [Crossref] [PubMed]
- Zheng YR, Lin SH, Chen YK, et al. Application of metagenomic next-generation sequencing in the detection of pathogens in bronchoalveolar lavage fluid of infants with severe pneumonia after congenital heart surgery. Front Microbiol 2022;13:954538. [Crossref] [PubMed]
- Tamma PD, Aitken SL, Bonomo RA, et al. Infectious Diseases Society of America Guidance on the Treatment of AmpC β-Lactamase-Producing Enterobacterales, Carbapenem-Resistant Acinetobacter baumannii, and Stenotrophomonas maltophilia Infections. Clin Infect Dis 2022;74:2089-114. [Crossref] [PubMed]
- Diao Z, Han D, Zhang R, et al. Metagenomics next-generation sequencing tests take the stage in the diagnosis of lower respiratory tract infections. J Adv Res 2022;38:201-12. [Crossref] [PubMed]
(English Language Editor: L. Huleatt)