HIF-1α siRNA reduces retinal neovascularization in a mouse model of retinopathy of prematurity
Retinopathy of prematurity (ROP) is the leading cause of childhood blindness in low birth weight and premature children, which is highly related to the introduction of oxygen therapy. In addition, ROP is a biphasic disease consisting of an initial phase of hyperoxia that induces irreversible damage to immature retinal vessels of the neonate, resulting in retinal ischemia, followed by a second phase of hypoxia-induced pathological neoangiogenesis (1,2). Although retinal angiogenesis is a fundamental element in the pathogenesis of blinding ROP (3,4). Studies have shown that blood vessel formation is the result of multiple growth factors, including the vascular endothelial growth factor, basic fibroblast growth factor, transforming growth factor-ß (TGF-ß), interleukin-1 (IL-1), IL-6, monocyte chemoattractant protein-1 (MCP-1) and tumor necrosis factor-α (TNF-α) (5). Among them, VEGF is most closely related with blood vessel formation and involved in the pathologic blood vessel formation that characterizes oxygen-induced retinopathy (OIR) and other proliferative retinopathies (6,7). In the first phase of ROP, supplemental oxygen suppresses VEGF production, thereby interfering with normal vascular development. In the second phase, which follows oxygen-induced vessel loss and subsequent hypoxia, retinal VEGF expression is induced, resulting in pathological neovascularization. Intravitreal injection of VEGF inhibitors during this phase decreases the neovascular response, indicating that VEGF is a critical factor contributing to retinal vascularization (8,9,10), and inhibition of the VEGF signaling pathway has been proved as an effective way to inhibit angiogenesis (11,12).
In the retina, the hypoxic condition increases the stability of the inducible subunit of the transcription factor hypoxia-inducible factor (HIF), HIF-1α (13). HIF-1α accumulation leads to the subsequent transactivation of HIF which, in turn, upregulates the expression of a variety of genes, including angiogenic growth factors (14). It was reported that HIF-1α can induce the expression of VEGF in hypoxic tissues (15,16).
siRNA has recently emerged as a very useful therapeutic method that suppresses the gene expression through the introduction of double-stranded RNA (17). An attractive advantage for using siRNA is its ability to temporally knockdown the expression of target genes specifically and potently, with little immunogenicity compared with those protein-related agents. In order to achieve a long-term gene suppression, the consecutive siRNA expression is required, which can be mediated by recombinant virus or eukaryotic expression plasmids such as pSUPER system (18). In our previous studies, we have created plasmids PSUPERH1-siHIF-1α that contained small fragments of HIF-1α RNA, and effectively inhibited the expression of VEGF mR-NA and HIF-1α proteins in vascular endothelial cells transfected with pSUPERH1-siHIF-1α (19,20).
Since few studies are available on the relationship between the VEGF signaling pathway and the occurrence of ROP, this study is designed to establish a mouse model of ROP by intravitreal injection with pSUPERH1-siHIF-1α to observe the change in VEGF and HIF-1α expression using western blotting and the progression of retinal angiogenesis, providing evidence for clinical treatment of ROP.
Materials and methods
Materials
The following materials were used in this study: C57BL/6J mice provided by Shanghai Experimental Animal Center of Chinese Academy of Sciences; cationic liposomes (LipofectamineTM 2000, LF2000) purchased from Invitrogen; goat anti-VEGF polyclonal antibodies purchased from R&D; goat anti-HIF-1α polyclonal antibody purchased Santa Cruz; and mouse anti-β-actin monoclonal antibody and FITC-Dextran (MW 2×106) purchased from Sigma.
Creation of pSUPERH1-siHIF-1α eukaryotic expression vector
The small interfering RNA target was designed according to the sequence of HIF-1α gene (NM_001530) in the human gene pool, with the target sequence of AAGAGGTGGATATGTCTGG (1214-1232). The oligonucleotide sequences of 64nt chemically synthesized sh-HIF-1α (HIF-1α small hairpin RNA) were: sense strand: 5'-GATC-CCCAAGAGGTGGATATGTCTGGTTCAAGAGACCAGA-CATATCCACCTCTTTTTTTGGAAAA, antisense strand: 5'-AGCTTTTCCAAAAAAAGAGGTGGATATGTCTGGTCTC-TTGAACCAGACATATCCACCTCTTGGG. The sense and antisense strands of sh-HIF-1α were annealed to form a double-stranded template. Double digested with Hind III and Bgl II, the linearized pSUPER.retro plasmids were connected to the annealed double-stranded in vitro and subject to transformation and screening for positive clones. The reorganized pSUPERH1-siHIF-1α vectors were then extracted and assessed by sequencing for the accuracy of the connection between the sh-HIF-1α oligonucleotide template and the pSUPERH1-siHIF-1α vectors.
ROP modeling and intravitreal injection with pSUPER-siHIF-1α
Forty-eight newborn C57BL/6J mice were randomly divided into the control and experimental groups (n=24 apiece) to create the model of ROP following the methods established by Smith and et al. (21). On day 7 after birth (P7), the mice were put together with their mother into an oxygen incubator at an oxygen concentration of (75±2)%. Five days later, the newborn mice (P12) and mother were transferred to a normal oxygen concentration. On P12, they received intraperitoneal injection with 30 mg/kg of 1% pentobarbital sodium for anesthesia. A micro-injector was then inserted 0.5 mm posteriorly of the limbus of each mouse under the microscope for intravitreal injection with 1 µL liposome-plasmid mixture (1 µL 500 ng/µL pSUPERH1-siHIF-1α: 1 µL LF2000). Meanwhile, mice in the control group were injected with empty vectors. Six mice in each group were sacrificed 12 h after the injection for detection of retinal HIF-1α (20); the other eight were killed on P17 for detection of retinal VEGF, FITC-Dextran fluorescence and nucleus count, respectively.
Western blotting of HIF-1α and VEGF expressions in two groups of mice
The animals were sacrificed 12 h after injection (P12). Their retinas were removed and put into a 1.5 mL EP tube with 100 µL tissue lysate, ground on ice for 30 min, and centrifuged at 14,000 rpm, 4 °C for 10 min. The protein concentration was measured with the BCA protein assay kit. Denatured proteins of 20 µg per hole were subject to 10% SDS-PAGE gel electrophoresis. The proteins were then transferred to the PVDF membrane under constant pressure, fixed with 2.5% bovine serum albumin for 1 h, and incubated 4 °C overnight with the addition of diluted HIF-1α (1:100), VEGF-A (1:500) and ß-actin (1:4,000), respectively. The membrane was washed with PBST for three times and added with the corresponding 1:10,000 HRP marked IgG. After incubated for 1 h, the membrane was washed with PBST again for three times. The target band was identified with ECL chemiluminescence detection in a dark room. Following Kodak X-ray film exposure, developing and fixing, the films were scanned and imported into the Eagle Eye II analyzer for gray-scale analysis and calibration against ß-actin as internal control to calculate the relative amount of target protein expression. Method of calculation: relative expression of the target protein = gray value of the target protein band / gray value of the ß-actin band.
FITC-Dextran fluorescence and nucleus count of new retinal vascular endothelial cells
After intraperitoneal administration of 1% sodium pentobarbital 30 mg/kg, P17 mice (six per group) were subject to open-chest surgery for left ventricular perfusion with 0.5 mL FITC-Dextran (50 mg : 1 mL 4% paraformaldehyde) using a 1 mL syringe. Their eyes were then rapidly removed. Under the operating microscope, the eyes were immersed in 1× PBS and cut along the limbus to remove the anterior tissues and the lens. Each retina was carefully separated using a self-made glass rod, cut radially, spread on the filter paper, immersed in 4% paraformaldehyde for 5 min. It was then stripped off and laid on a clean glass slide, paved, added with 2% gelatin drops, covered with a coverslip, placed under the fluorescence microscope and photographed.
The mice eyes were fixed with 4% paraformaldehyde, dehydrated through an ethanol gradient, embedded in paraffin, and cut into serial sagittal sections through the cornea parallel to the optic nerve (6 µm thick). Eight random sections of each eye, not containing the optic nerve, were processed by hematoxylin-eosin (HE) staining. The number of nuclei in vascular endothelial cells protruding the internal limiting membrane was counted under a microscope. The average endothelial cell count was used to describe the number of nuclei per eye in statistical analysis (21).
Statistical analysis
The analysis was completed in SPSS 11.0. All results were expressed as mean ± standard deviation (x±s). Measurement data were taken into one-way analysis of variance (ANOVA). Differences between groups were compared using Dunnett-t test, with a P value of less than 0.05 being considered statistically significant.
Results
HIF-1α and VEGF expressions in two groups of mice
The experiment group was associated with significantly less expression of retinal HIF-1α and VEGF proteins compared with the control group (90% and 65% lower, P<0.01) (Figures 1,2).
Retinal fluorescence of the two groups
The control group was associated with irregular expansion of large, tortuous retinal blood vessels with peripapillary capillary occlusion; a large non-perfusion area was seen. The surrounding normal capillary network had disappeared and a cluster of new blood vessels with fluorescein leakage was visible. In the experiment group, the structure of the retinal vascular network remained clear, and the central retinal vascular tortuosity and irregular expansion was significantly milder when compared with the control group. The capillary non-perfusion area around the optic disc was significantly reduced, peripheral capillary network basically normal, and there was remarkably fewer angiogenesis (Figure 3).
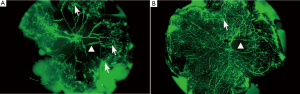
Neovascular endothelial cell count
The number of retinal vascular endothelial cells protruding the internal limiting membrane was 48±5 in the control group and 18±5 in the experiment group, the difference being statistically significant (P<0.001) (Figures 4,5).
Discussion
The animal model of retinopathy of prematurity established by Smith and et al. follows a similar pathogenetic mechanism in humans. Seven days after birth, great retinal vessels are basically developed while capillaries are still immature in mice, where a high-oxygen environment can cause spasm of these immature retinal vessels. Returned to normal oxygen environment after 5 days, the retina is relatively hypoxic and triggers expansion of blood vessels to compensate at the early stage. When decompensation occurs later, formation of new blood vessels will be the result. The mouse model of ROP is mainly characterized by a large ischemic central retinal non-perfusion area and retinal neovascularization, while the ischemic non-perfusion area is observed in the peripheral retina in humans.
As shown in this study, intravitreal injection of a small amount of pSUPERH1-siHIF-1α could effectively inhibit retinal neovascularization in the mouse model. The following factors were probably involved in this process: (I) After injected intravitreally, pSUPER (H1-siHIF) may successfully enter into the “target” retinal cells that produced HIF-1α. Studies showed that increased HIF-1α expression was mostly present in the inner retinal tissue, where retinal ganglion cells were located, in the ROP mousemodel. Plasmids wrapped in intravitreally injected cationic liposomes could easily disseminate in the vitreous cavity, penetrate the retinal internal limiting membrane, spread in the retinal tissue, and eventually be absorbed by retinal ganglion cells with continued expression for as long as one month (22). Therefore, by entering and affecting the related retinal cells, intravitreally injected pSUPERH1-siHIF-1α could cut off the “source” of HIF-1α production. (II) Amplification of RNA interference: Uptaken by retinal cells, pSUPERH1-siHIF-1α produced the targeted HIF-1α siRNA in cells by transcription. On the one hand, siRNA caused degradation of HIF-1α mRNA in retinal cells by binding with intracellular ribozyme complexes; on the other hand, the targeted HIF-1α siRNA could serve as primers to produce the complementary strand for HIF-1α mRNA using intracellular HIF-mRNA as a template through the effect of RNA-directed RNA polymerases (RdRP). As a result, HIF-1α mRNA became a double-stranded RNA. The newly synthesized double-stranded RNA was cleaved into a new targeted HIF-1α siRNA under the action of enzyme Dicer in retinal cells (23). Through that polymerase chain reaction, HIF-1α siRNA had a significant increase in number, and as a result, intravitreal injection of a small amount of pSUPERH1-siHIF-1α could impose a great inhibitory effect on retinal neovascularization.
A nuclear protein with transcriptional activity generated in hypoxic cells, HIF-1 could bind with target genes (such as VEGF) to regulate transcription and post-transcriptional processes, triggering the body’s adaptive response to hypoxia and ischemia (24). VEGF, also known as the vascular permeability factor, was the specific mitogen for vascular endothelial cells. It played an important role for angiogenesis by enabling deformation, movement, division and proliferation of the cells and increasing vascular permeability. Previous studies showed increased expression of VEGF in a variety of retinal vascular diseases, which was a key factor of retinal neovascularization (25,26). Ozaki and et al. (25) found that in retinopathy of prematurity in mice, hypoxia induced higher expression of retinal HIF-1, which in turn promoted the expression of its downstream gene VEGF. This study showed that suppression of the HIF-1 expression by RNAi could down-regulate retinal expression of VEGF, thereby inhibiting the occurrence of retinal neovascularization. This suggested that the VEGF expression was highly related to HIF-1 in retinopathy of prematurity, and inhibiting the expression HIF-1 could reduce retinal neovascularization.
In summary, as shown in this study, downregulation of HIF-1 expression by RNA interference could effectively reduce the incidence of retinal neovascularization in the mouse model of ROP. That might be used as a new therapeutic option for the clinical treatment of ROP.
Acknowledgements
None.
Footnote
Conflicts of Interest: The authors have no conflicts of interest to declare.
References
- Fleck BW, McIntosh N. Pathogenesis of retinopathy of prematurity and possible preventive strategies. Early Hum Dev 2008;84:83-8. [PubMed]
- Brummelkamp TR, Bernards R, Agami R. A system for stable expression of short interfering RNAs in mammalian cells. Science 2002;296:550-3. [PubMed]
- An international classification of retinopathy of prematurity. The Committee for the Classification of Retinopathy of Prematurity. Arch Ophthalmol 1984;102:1130-4. [PubMed]
- Li QP, Wang ZH, Chen YQ, et al. Bedside treatment of retinopathy of premeturity by laser photocoagulation. China J Contemp Pediatr 2010;12:693-5.
- Simó R, Carrasco E, García-Ramírez M, et al. Angiogenic and antiangiogenic factors in proliferative diabetic retinopathy. Curr Diabetes Rev 2006;2:71-98. [PubMed]
- Chen J, Smith LE. Retinopathy of prematurity. Angiogenesis 2007;10:133-40. [PubMed]
- Penn JS, Madan A, Caldwell RB, et al. Vascular endothelial growth factor in eye disease. Prog Retin Eye Res 2008;27:331-71. [PubMed]
- Andreoli CM, Miller JW. Anti-vascular endothelial growth factor therapy for ocular neovascular disease. Curr Opin Ophthalmol 2007;18:502-8. [PubMed]
- Kusaka S, Shima C, Wada K, et al. Efficacy of intravitreal injection of bevacizumab for severe retinopathy of prematurity: a pilot study. Br J Ophthalmol 2008;92:1450-5. [PubMed]
- Guma M, Rius J, Duong-Polk KX, et al. Genetic and pharmacological inhibition of JNK ameliorates hypoxia-induced retinopathy through interference with VEGF expression. Proc Natl Acad Sci U S A 2009;106:8760-5. [PubMed]
- Robinson GS, Pierce EA, Rook SL, et al. Oligodeoxynucleotides inhibit retinal neovascularization in a murine model of proliferative retinopathy. Proc Natl Acad Sci U S A 1996;93:4851-6. [PubMed]
- Bainbridge JW, Mistry A, De Alwis M, et al. Inhibition of retinal neovascularisation by gene transfer of soluble VEGF receptor sFlt-1. Gene Ther 2002;9:320-6. [PubMed]
- Bartoli M, Al-Shabrawey M, Labazi M, et al. HMG-CoA reductase inhibitors (statin) prevents retinal neovascularization in a model of oxygen-induced retinopathy. Invest Ophthalmol Vis Sci 2009;50:4934-40. [PubMed]
- Semenza GL. Hydroxylation of HIF-1: oxygen sensing at the molecular level. Physiology (Bethesda) 2004;19:176-82. [PubMed]
- Rosmorduc O, Housset C. Hypoxia: a link between fibrogenesis, angiogenesis, and carcinogenesis in liver disease. Semin Liver Dis 2010;30:258-70. [PubMed]
- Westra J, Molema G, Kallenberg CG. Hypoxia-inducible factor-1 as regulator of angiogenesis in rheumatoid arthritis - therapeutic implications. Curr Med Chem 2010;17:254-63. [PubMed]
- Elbashir SM, Lendeckel W, Tuschl T. RNA interference is mediated by 21- and 22-nucleotide RNAs. Genes Dev 2001;15:188-200. [PubMed]
- Brummelkamp TR, Bernards R, Agami R. A system for stable expression of short interfering RNAs in mammalian cells. Science 2002;296:550-3. [PubMed]
- Xu HZ, Liu SZ, Xia XB, et al. The inhibition effect of HIF-1α siRNA on VEGF mRNA expression in vitro. Chinese Journal of Optometry and Ophthalmology 2007;9:228-31.
- Xiong Y, Xia XB, Xu HZ, et al. Inhibitory effect of interfering RNA targeting HIF-1α protein in mice retina. International Journal of Ophthalmology 2007;7:361-4.
- Smith LE, Wesolowski E, McLellan A, et al. Oxygen-induced retinopathy in the mouse. Invest Ophthalmol Vis Sci 1994;35:101-11. [PubMed]
- Masuda I, Matsuo T, Yasuda T, et al. Gene transfer with liposomes to the intraocular tissues by different routes of administration. Invest Ophthalmol Vis Sci 1996;37:1914-20. [PubMed]
- Lipardi C, Wei Q, Paterson BM. RNAi as random degradative PCR: siRNA primers convert mRNA into dsRNAs that are degraded to generate new siRNAs. Cell 2001;107:297-307. [PubMed]
- Takahashi R, Tanaka S, Hiyama T, et al. Hypoxia-inducible factor-1alpha expression and angiogenesis in gastrointestinal stromal tumor of the stomach. Oncol Rep 2003;10:797-802. [PubMed]
- Ozaki H, Yu AY, Della N, et al. Hypoxia inducible factor-1alpha is increased in ischemic retina: temporal and spatial correlation with VEGF expression. Invest Ophthalmol Vis Sci 1999;40:182-9. [PubMed]
- Frank RN. Diabetic retinopathy. N Engl J Med 2004;350:48-58. [PubMed]