Innovative interventional catheterization techniques for congenital heart disease
Transcatheter valve implantation
The first report of successful transcatheter pulmonary valve implantation was in 2000 (1). After a successful multi-center United States Investigational Device Exemption trial, the Melody™ Transcatheter Pulmonary Valve (Medtronic, Inc., Minneapolis, Minnesota, USA) became the first FDA approved transcatheter pulmonary valve in 2010 (2,3). Results from the United States Post-Approval Study and several international Melody valve implantation registries, led to the quick adoption of this therapy in patients with congenital heart disease as these valves exhibited excellent reduction in both right ventricular outflow tract stenosis and insufficiency (4-6). The initial indication for Melody valve implantation was for use in dysfunctional right ventricle-to-pulmonary artery conduits. However, off-label use of the device quickly expanded to dysfunctional bioprosthetic pulmonary valves (now an on-label use of the Melody valve), as well as implantation in non-pulmonary positions, including the mitral valve, tricuspid valve, and aortic valve, as well as implantation in the bilateral branch pulmonary arteries (7-10).
While the Melody valve, and its delivery system, were specifically designed for use in the pulmonary position, the Edwards Sapien valve (Edwards Lifesciences, Irvine, CA, USA) originally designed as a transcatheter aortic valve, also expanded its market into the pulmonary space (11). The next generation device, Edwards Sapien XT valve (Edwards Lifesciences, Irvine, CA, USA) received FDA approval in 2016 for the use in dysfunctional right ventricle to pulmonary artery conduits. The COMPASSION Trial (COngenital Multicenter trial of Pulmonic vAlve regurgitation Studying the SAPIEN InterventIONal THV COMPASSION Trial) is completing data collection and the COMPASSION Post-Approval Study is currently underway. A newer version of the Sapien valve, the S3 valve, has also been implanted successfully in the pulmonary position and non-pulmonary prosthetic valves (12-14).
A recent meta-analysis of implanted transcatheter pulmonary valves, including both Melody and Sapien valves, confirms what has been shown in multiple smaller studies: a very high rate of implantation success (96.2%) in over 1,000 reported implants in the literature (15). Further, after accounting for the practice of pre-stenting conduits, which has drastically lowered the incidence of stent fracture in the Melody valve population, re-intervention rates were quite low (2.9 per 100 patient years, compared to 6.9 per 100 patient years in patients without pre-stenting).
Despite the excellent success of transcatheter pulmonary valve therapies, patients with dysfunctional conduits or bioprosthetic valves comprise the minority of patients with right ventricular outflow tract dysfunction. It is estimated that the currently available therapies will only effectively treat ~15% of patients with severe pulmonary insufficiency (16). Of the remaining 85% of patients, the majority have severe pulmonary regurgitation secondary to surgical right ventricular outflow tract patch augmentation, typically in the setting of Tetralogy of Fallot. Over time, chronic pulmonary regurgitation leads to right ventricular dilation, decreased exercise tolerance, and increased risk for arrhythmia and sudden cardiac death (17-21). These patients, however, often have severe dilation of the outflow tract making pulmonary valve replacement with currently available transcatheter valves not feasible and therefore making surgical pulmonary valve replacement the only option. Now with the availability of larger diameter S3 valves, as well as several creative ways to reduce the size of the right ventricular outflow tract to allow for the safe implantation of currently available transcatheter valves (22,23), treatment of so-called “native” outflow tracts is possible but remains limited. Therefore, this patient population represents a significant unmet need in the field of congenital heart disease.
Several newer valves are currently being developed to expand our ability to treat patients with severe pulmonary insufficiency and right ventricular outflow tracts too large for currently approved devices. The first of such devices, which is now better known as the Medtronic Harmony™ Transcatheter Pulmonary Valve (Medtronic, Inc.), was successfully implanted in 2010 (16). This self-expanding nitinol covered stent with a porcine pericardial valve sewn at the center has shown promising results in animal models, with improved right ventricular size on follow-up volumetric imaging (24). More recently, the Harmony early feasibility study showed a high rate of implantation success (95%) with few procedural complications (25). Furthermore, 100% of patients had mild or less pulmonary regurgitation at 6-month follow-up, compared to 95% with severe and 5% with moderate pulmonary regurgitation at baseline (25). The Pivotal Trial for the Harmony valve is currently underway in the United States with successful implantation in the first patient in September 2017. Other devices being implanted outside the United States include the Venus P valve (Venus Medtech, Shanghai, China) (26-29), as well as the self-expanding device by the TaeWoong Medical Company (Gyeonggi-do, Republic of Korea) (30). All devices have shown promising early results but further longitudinal study is necessary to understand how each of these devices will change the landscape of pulmonary valve replacement.
Transcatheter valve implantation—conclusions
Transcatheter valve implantation is one of the fastest growing areas of innovation in our field. Current devices have allowed us to treat a large number of patients who previously required surgical re-operations and future technologies promise to grow the patient population that we can serve. A critical next step will be refining when we intervene, particularly in the setting of chronic pulmonary regurgitation, and understanding if earlier intervention improves long-term patient outcomes, especially compared to surgical valve replacement.
Hybrid procedures
A “hybrid procedure” refers to any procedure where interventional catheterization and surgical techniques are used in tandem. In congenital heart disease, hybrid procedures are often synonymous with the hybrid stage I palliation for infants with hypoplastic left heart syndrome (HLHS), however hybrid procedures are utilized in many other situations extending beyond infants with HLHS. In fact, in the era of increasingly complex interventions on increasingly high-risk patients, hybrid procedures offer many potential advantages over traditional interventional catheterization and surgical techniques because, during hybrid procedures, we are able to combine the best parts of each specialty. Often, performing a procedure with a hybrid approach allows for more direct access with larger bore equipment in smaller patients while avoiding cardiopulmonary bypass. More importantly, hybrid procedures are an example of using cross-discipline innovation, collaboration, and group-thought to solve complex problems.
The first “hybrid” procedure was described in 1972 (31). Although never referred to as a hybrid procedure, what we would now refer to as hybrid techniques were utilized to facilitate patent ductus arteriosus closure. Interestingly, the authors comment that they were “impressed by the simplicity and quickness of the procedure,” which is often a main advantage of hybrid procedures over more traditional surgical and transcatheter techniques (31). Since that time, hybrid procedures have been used for a myriad of purposes (Figure 1). Here we will highlight two of these hybrid procedures; the hybrid approaches for hypoplastic left heart syndrome and pulmonary atresia with intact ventricular septum.
HLHS
Probably the most commonly discussed hybrid procedure in the field of pediatric cardiology is the use of a hybrid procedure for patients with HLHS. This alternative to the standard surgical Norwood palliation involves stenting of the ductus arteriosus, bilateral pulmonary artery band placement, and atrial septostomy, and was first described in 1993 (32,33). Now commonly referred to as the hybrid stage I palliation, or the hybrid Norwood, the procedure has undergone some modifications with time but the three main objectives of the hybrid stage I palliation for infants with HLHS are the same as the surgical approach: create unobstructed pulmonary return across the atrial septum, create unobstructed systemic arterial blood flow, and create restricted pulmonary blood flow (Figure 2).
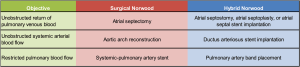
From a technical standpoint, the hybrid stage I palliation can be completed by a surgeon and interventional pediatric cardiologist with minimal additional equipment or training. Several techniques have been developed to help ease the learning curve associated with the procedure, like the appropriate tightness when placing the pulmonary artery bands (34). Some centers across the country have adopted a strategy of using the hybrid Norwood in all single ventricle patients, while other centers use the hybrid Norwood only in high-risk patients, or patients felt to be at a higher risk of morbidity or mortality after the surgical Norwood palliation (35-42). In these high-risk patients, avoiding cardiopulmonary bypass during the neonatal period may lower mortality and morbidity during the staged single ventricle palliation (43-48). Our center is currently examining the impact of utilizing the hybrid approach in high-risk single ventricle patients when compared to a similar high-risk cohort prior to the advent of the hybrid procedure.
Importantly, the hybrid stage I palliation has also been used successfully in the non-single ventricle population. For example, in patients with factors which might place them at higher risk of morbidity or mortality after major complex congenital heart repair during infancy, the stage I hybrid palliation can be used to stabilize the circulation and post-pone definitive anatomic repair until later in childhood (49,50).
There are some anatomic and potential pathophysiologic complications uniquely associated with the hybrid stage I palliation, including recurrent atrial septal restriction, reverse coarctation of the aorta, and pulmonary artery stenosis. Additionally, several studies comparing the hybrid to standard surgical Norwood approaches using computational flow modeling have found some important differences in systemic and cerebral oxygenation as well as ventricular mechanics which may favor the standard surgical approach (51,52). Therefore, as with any novel approach to a complex problem, careful and longitudinal analysis will be important as we identify which patients will most benefit from the hybrid palliation.
Pulmonary atresia with intact ventricular septum
A subset of patients with pulmonary atresia with an intact ventricular septum will be anatomically suitable to undergo a biventricular repair. This involves opening the right ventricular outflow tract, typically in the neonatal period. Traditionally, this could be done via a surgical approach (pulmonary valvotomy) or via a transcatheter approach (percutaneous pulmonary valve perforation and balloon valvuloplasty). The hybrid procedure in this lesion is a great example of how taking advantage of the benefits from each approach facilitates a procedure while minimizing procedural complications and long-term deleterious effects.
The hybrid approach to pulmonary atresia with intact ventricular septum typically involves periventricular access via a midline sternotomy with needle perforation of the atretic pulmonary valve followed by balloon pulmonary valvuloplasty (53,54). In many patients, a systemic-pulmonary artery shunt is performed due to persistent (typically muscular) subvalvar right ventricular outflow tract obstruction. Fortunately, this shunt can be placed without the use of cardiopulmonary bypass. Surgical exposure to the right ventricle allows for direct access to the pulmonary valve with much lower risk of right ventricular outflow tract perforation, a risk of the transcatheter approach to pulmonary valve perforation. Combining the use of transcatheter techniques, the entire procedure can be performed without cardiopulmonary bypass with nearly identical high procedural success, low procedural complication rates, and mid-term outcomes compared to the surgical approach (53). Proponents of the transcatheter approach frequently site the need for a midline sternotomy as a major downfall of the hybrid approach. However, up to 76% of patients (range, 33–76%) who undergo the transcatheter approach ultimately require a midline sternotomy either to augment pulmonary blood flow (typically with placement of a systemic-pulmonary artery shunt) in the setting of post-procedure hypoxia, or due to inadvertent perforation of the right ventricular outflow tract (55-61). A comparison of the various approaches for right ventricular decompression in patients with pulmonary atresia with intact ventricular septum is detailed in Figure 3.
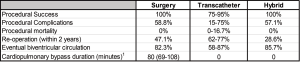
Hybrid procedures—conclusions
As we continue to develop less invasive ways to treat complex congenital heart disease, hybrid procedures will undoubtedly continue to help shape the interventional landscape. We must continue to both push the boundaries of these procedures, while continuing to study the outcomes to assure we are providing acute results better than standard transcatheter and surgical approaches alone, or acute results equivalent to standard approaches with better overall long-term patient outcomes. For some types of hybrid procedures, like intra-operative pulmonary artery stent placement, fairly long-term patient follow-up is available (62). Only by directly comparing hybrid approaches to other standard approaches will we be able to choose the best options for our patients.
Biodegradable technologies
Through years of innovation, interventional pediatric cardiologists have been afforded a wide array of devices to palliate and treat patients with congenital heart disease. These include stents of various diameters, lengths, and physical properties, coils and vascular occlusion devices, and devices designed to close atrial septal defects, ventricular septal defects, and the patent ductus arteriosus. However, a significant drawback of all these devices is their permanence. Outside of surgical excision, these devices are implanted permanently. For some patients, there are potential benefits to permanent implantation. For example, left pulmonary artery stenosis secondary to compression by the aorta likely will require a permanent stent to maintain patency. But a major consideration for the majority of patients with congenital heart disease undergoing cardiac catheterization procedures early in life is somatic growth. A stent placed in the aorta or pulmonary artery at 8 years of age will undoubtedly require further dilation in adulthood. And besides somatic growth, many heart lesions would be much better treated with a temporary implant. For example, at the current time closure of a patent ductus arteriosus or atrial septal defect requires implantation of a device (coil, vascular occlusive device, septal occlude, etc.) which generally results in immediate and complete occlusion. But in a relatively short time period, endothelialization forms a permanent “natural” covering over the defect. At this point, the implanted device, which really served as a scaffold to facilitate the endothelialization process, is an unnecessary remnant of the procedure. So how can we get the excellent results we expect from our current medical devices but limit the negative consequences related to their permanence? One possible solution may come in the form of biodegradable technology. Biodegradable materials have been used for years in medicine (for example, absorbable sutures), however, more recently, there has been tremendous effort by several research teams to develop these materials for use in patients with congenital heart disease. This includes development of vascular stents and occlusion devices, which can be applied across a wide variety of cardiovascular diseases.
Grossly, current biodegradable materials can be broken into two major categories based on their composition: bioabsorbable polymers and biocorrodible metals (63). Each material has advantages and limitations and each may have an important role in various devices. Polymers, for example poly L-lactic acid, break down by hydrolysis. The material strength and rate of bioabsorption can be altered by engineering the composition of the individual monomers and how they are inter-connected (63,64). Because bioabsorption occurs by hydrolysis and the monomers are commonly occurring and/or inert substances, these are likely to be very safe. One drawback, especially from the perspective of the interventional cardiologists, is that these materials are completely radiolucent unless modified. In contrast, biocorrodible materials, like zinc or magnesium, break down by biocorrosion. Biocorrosion is potentially inflammatory or toxic at the cellular or tissue level, although some research indicates Zinc may actually suppress inflammation at the cellular level. For the development of stents, this may be beneficial in lowering rates of neointimal proliferation, which is problematic for current small and medium diameter bare metal stents (65,66). Being metallic, there are radio-opaque and may have more similar physical properties, including radial strength and deployment characteristics, to currently commercially approved medical devices.
Many biodegradable stents and occlusion devices are in various stages of development with potential applications within the congenital heart disease space (63,65,67). The first commercially approved bioabsorbable stent became available in the United States in July 2016. The Absorb GT1 Bioresorbable Vascular Scaffold (BVS) stent (Abbott Vascular, Abbott Park, IL, USA), showed promise for use in coronary artery disease during the initial ABSORB trials (68). This bioabsorbable polymer stent made of poly L-lactic acid, was compared directly to a commercially available bare metal coronary stent through a series of first in man, then prospective and randomized clinical trials (68). Unfortunately, the stent is no longer commercially available. Although the company cites low sales, the device was removed from the market in mid 2017 due to a higher incidence of late stent thrombosis, in addition to evidence of higher rates of target lesion failure during long-term follow-up from the ABSORB III clinical trial (69). Although this is certainly a major set-back for biodegradable technologies, hopefully this early failure does not hinder future research and development of what will surely be an important part of the pediatric interventionalists’ armamentarium in the near future. Most recently, in Europe a new device for atrial septal defect closure has received CE mark. The Carag bioresorbable septal occluder (CBSO) (Carag AG) was approved for use across Europe in mid-September 2017 (Figure 4). With time, we hope that this device and other devices of its kind will continue to improve the care we provide to patients with congenital heart disease.
Biodegradable technologies—conclusions
Biodegradable technologies represent an important merger between materials scientists and clinicians as we strive to improve the care we deliver with both excellent short-term, and long-term outcomes for our patients. In congenital heart disease, accounting for growth potential is crucially important as we perform interventions on increasingly small children and strive to minimize re-interventions on devices implanted during childhood.
Magnetic resonance imaging-guided cardiac catheterization
X-ray fluoroscopy has traditionally provided guidance for cardiac catheterization procedures, however fluoroscopy is limited by 2-dimensional projections, limited soft tissue definition, and necessary exposure to ionizing radiation. In patients with congenital heart disease, early and multiple exposures to ionizing radiation over the course of their lives puts them at higher cumulative risk associated with ionizing radiation exposure (70-73). Practices and techniques to minimize radiation exposure in this vulnerable group of pediatric patients, therefore, is of particular focus in pediatric interventional cardiology. One emerging technology that allows for minimal or no radiation exposure is magnetic resonance imaging (MRI)-guided cardiac catheterization.
Cardiac MRI (CMR) provides excellent soft-tissue definition and provides important information about structure, function, and perfusion/flows. However, measurement of intracardiac hemodynamics still requires cardiac catheterization, which is invasive and exposes patients to radiation. The guidance for this catheterization can be modified to include no radiation through the use of real-time CMR. Clinical CMR was first reported as an adjunct to X-ray fluoroscopy in adults over 10 years ago (74) and has continued to evolve since that time, with many small studies evaluating the feasibility and outcomes of radiation free CMR-guided cardiac catheterization. This includes both animal and adult pilot studies for both diagnostic and limited interventional procedures (75-77). In their pilot study comparing X-ray and CMR-guided right heart catheterization in 16 adult patients, Ratnayaka and his colleagues found that CMR-guided catheterization was able to be safely and successfully performed, and had a total procedure time similar to that of X-ray guided catheterization (76). In their patients, CMR-guided catheterization has now become the preferred modality for adult patients referred for a right heart catheterization. They have since published on 50 radiation-free transfemoral CMR-guided right heart catheterizations in children (78). All catheterizations were successful with no complications, and given the short procedure time with a realistic workflow, they hope to be able to offer this technology routinely in the pediatric population in the near future (78).
CMR-guided catheterization requires an elaborate set-up, which has limited its widespread use. A combined fluoroscopy/CMR suite with biplane X-ray fluoroscopy and adjoining CMR rooms, separated by X-ray and radiofrequency shielded doors, are fashioned with special mobile tables that can transfer a patient from the fluoroscopy lab to the MRI scanner while maintaining sterility (Figure 5). The other challenge with CMR-guided catheterization is that all equipment used in the MRI room must be MRI compatible, including all catheters, guidewires, and potential devices. At this time, visualization of catheters on MRI is limited to the tip only, where a commercially available balloon tipped catheter is inflated with gadolinium to allow localization of the catheter tip. There are currently no commercially available guidewires to aid manipulation of catheters into specific locations or over which balloons and stents can be delivered, and this remains the biggest hurdle to the expansion of CMR-guided interventional catheterization. The added superiority of real-time MRI imaging, however, has the potential to allow for safer performance of complex interventions. Recently, Ratnayaka et al. published their experience with MRI-guided transcatheter cavopulmonary shunt creation in 15 swine (79).
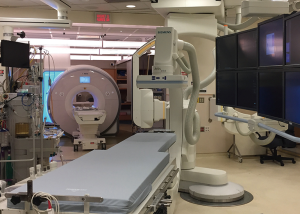
Magnetic resonance imaging-guided cardiac catheterization—conclusions
The ability to offer transcatheter cardiac procedures more safely and without ionizing radiation is particularly relevant for patients with complex congenital heart lesions who require multiple catheterization procedures over their lifetime. Although eliminating exposure to ionizing radiation, and continuing to expand the scope of transcatheter interventions, seems like a pipe dream, it may soon become a reality with CMR-guided catheterization.
Innovative imaging and modeling techniques
No transcatheter intervention would be possible without appropriate imaging to plan, guide, and assess the impact of a procedure. The development and more widespread use of innovative imaging techniques is paramount in the interventional cardiologist’s ability to perform increasingly complex interventions on complex anatomy, broadening the scope of procedures that can be performed via a transcatheter rather than surgical approach. Three-dimensional (3D) imaging and advanced visualization techniques have allowed for superior imaging with lower radiation doses and have allowed for the understanding of more complex anatomy and anatomic relationships.
3D rotational angiography (3DRA)
Originally designed for use in interventional neurovascular procedures (80,81), over the last 10 years, angiographic computed tomography (CT), or 3DRA, has become an integral part of the modern day cardiac catheterization lab. This technology uses cross-sectional CT images created from a rotational angiogram to display a volumetric data set. The angiogram is performed over a slow, multiple second injection of dilute contrast (typically 50–60% strength) while a standard C-arm mounted flat panel detector rotates ~190 degrees around the patient. This rotational angiogram provides a robust data set, which can be post-processed into a 3D reconstruction within minutes. The source images obtained are identical to that of a cross-sectional CT scan, therefore in addition to post-processing into a 3D reconstruction, the cross-sectional CT images may also be viewed and are particularly useful for visualizing soft tissue structures, allowing for the operator to appreciate relationships and interactions between vascular/cardiac structures and other soft tissue structures within the chest (82,83).
Respiratory and cardiac motion over the duration of the rotational angiogram make the cardiac applications of this technology understandably more difficult than the neurovascular applications for which they were originally designed. However 3DRA can provide diagnostic quality images in the majority of cases with comparable overall radiation and contrast doses to standard cineangiography (84-87). The 3D reconstructions are particularly useful in understanding complex pulmonary artery or aortic arch anatomy and the relation of these vascular structures to soft tissue structures such as the airway (82,83), frequently providing additional, clinically relevant data when compared with standard biplane angiography (87). While dependent in part on the structure being visualized, quantitative measurements of structures made by 3DRA are comparable to those by 2D angiography (88). The reconstruction can also be manipulated on the workstation to visualize areas that are difficult or impossible to profile by standard 2D angiographic views and can be more sensitive for detection of some stenotic lesions (88). “Ideal” camera angles for viewing a particular lesion can also be easily identified, standard fluoroscopic C-arms moved to these angles, and the lesion of interest viewed at optimal working 2D angles. This can potentially lower the number of angiograms needed to visualize a lesion, which may result in lower radiation exposure and contrast dose. An overlay of the reconstruction can also be projected on the fluoroscopic image to allow for real time guidance of catheters and interventional equipment and can assist with complex catheter manipulations (Figure 6).
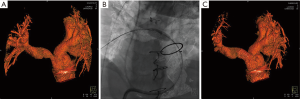
3DRA—conclusions
The use of 3DRA in congenital heart disease allows for a more robust understanding of complex anatomic relationships compared to standard biplane angiography. The ability to create and manipulate 3D volumetric data sets in real time allows for facile evaluation of both cardiac and non-cardiac lesions and procedural guidance. These attributes make 3DRA an important adjunctive imaging modality for the treatment of complex congenital heart disease in cardiac catheterization procedures.
Multimodality image fusion
While 3DRA allows for acquisition of a new CT dataset during the catheterization, if a pre-existing cross-sectional dataset derived from CMR or CT scan is available, these images can be fused with live fluoroscopy to provide additional anatomic guidance during a cardiac catheterization. Particularly appealing, the use of X-ray magnetic resonance fusion (XMRF) allows a 3D dataset with soft tissue definition obtained without ionizing radiation to be used to guide a live catheterization. Various methods can be used to fuse these images with fluoroscopy by using internal (bone, airway, artifact, calcium) or external fiducial markers (89,90). Once fused, these overlays can be used similarly to those obtained with 3DRA, and function to guide catheter manipulation, choose optimal working camera angles, and guide interventions. Multiple small studies have shown that use of XMRF can reduce radiation and contrast dose and reduce fluoroscopic time for select interventional procedures (89,91,92)
The multimodality fusion techniques described above have the downside that they do not provide real-time imaging to account for cardiac and respiratory motion and changes in patient position. A newer fusion technique, initially approved for use in 2012, is a software called EchoNavigator (Philips Healthcare, Best, The Netherlands), which allows for fusion of real-time echocardiographic images onto live fluoroscopy (93). This technique takes the wide field of view and excellent visualization of bony structures, devices, and catheters provided by fluoroscopy, and couples it with the excellent soft tissue visualization provided by echocardiography (standard 2D or 3D). These images are then displayed in the same orientation as a single fused image viewable by the operator (93). Areas of interest, such as a baffle or paravalvular leak, can be marked on the echo image and then viewed on the fused fluoroscopy image to help with localizing structures not visible by fluoroscopy alone. This modality has found wider use in adult structural heart disease, with multimodality guidance utilized for left atrial appendage occlusion, transcatheter mitral valve repair, and paravalvular leak closure (93-96). It has started to find a place within the realm of congenital heart disease as well, with proposed uses for atrial and ventricular septal defect closure, Fontan fenestration closure, and transseptal puncture (97,98). Compared to a historical control group of patients undergoing atrial septal defect closure, use of EchoNavigator was shown to provide superior procedural guidance compared with standard echocardiography alone in the majority of cases, with lower overall fluoroscopy time and radiation dose (97).
Multimodality image fusion—conclusions
Fusion of CT or MRI derived volumetric data sets, and 2D/3D echocardiographic data sets with fluoroscopy allows for improved understanding of anatomic relationships and enhanced procedural guidance. While the use in congenital heart disease is in its infancy, we anticipate continued growth in this area as we continue to look for ways to lower radiation doses and await application of additional technologies, like 3D TEE, in the pediatric space.
3D printing and 3D modeling
Over the last decade, advances in 3D imaging techniques have allowed the ability to translate cross sectional imaging data into interactive, real-life modeling through the use of 3D printing (99). Also known as rapid prototyping or additive printing, 3D printing has been used since the 1980s. More recent advances in imaging and printing technology have allowed for 3D printing to be more readily accessible (cheaper and faster) and used in dynamic cardiac structures (100). Non-invasive imaging datasets (CT, MRI, and more recently 3D echocardiography) can now be used to create patient-specific 3D printed heart models of complex cardiac anatomy and provides an innovative approach to pre-procedural planning, communication, and patient and trainee education (99,101) (Figure 7).
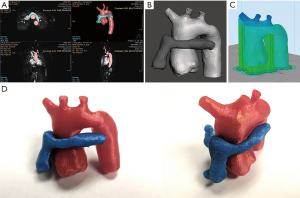
Historically, cross sectional imaging in the form of contrast-enhanced CT images were used most frequently to create 3D models. Free-breathing 3D whole heart MRI has become increasingly popular with complex congenital heart lesions (102). More recently, 3D echocardiography has also been utilized for the creation of 3D printed models. This modality offers a more accessible and cost-effective option and is particularly beneficial when visualization of structures not well seen by cross-sectional imaging, such as the atrial septum and valve leaflets, are the area of interest (103-105). Hybrid imaging, which combines a cross sectional dataset with an ultrasound dataset to take advantage of the best components of multiple modalities, is also evolving and is a promising avenue for production of a complete heart model with imbedded valve leaflets (106,107).
Once created, these models have been used successfully in the understanding of intracardiac and extracardiac lesions including complex intracardiac relationships, aortic arch anomalies, pulmonary artery branches, and aortopulmonary collaterals. These models can then facilitate pre-procedural planning both for transcatheter and surgical interventions. Multiple studies have shown the feasibility and accuracy of 3D printed models for reconstruction of complex cardiac lesions, with excellent correlation (within millimeters) of 3D models to the anatomic details visualized by cross-sectional imaging or by direct visualization in the operating room (104,105,108-110). In interventional cardiology, these models can be used in pre-procedural planning, in order to better understand complex anatomy (104,111-114) and to allow for mock-implants of a device or stent within a 3D printed model to better understand device fit in a particular patient (113,115,116). Additionally, there is the potential for patient specific 3D models to be used for education of patients and families, improving patient satisfaction, and improving communication between providers and between providers and their patients.
While there is endless potential for the use of 3D printing within congenital heart disease, there are a number of limitations. There is currently a lack of a standardized approach to imaging, segmentation, and processing which results in wide variation in the models produced. While the cost and time associated with segmentation and printing has decreased significantly over the recent years, these are still very real limitations to wide-spread use of the technology (101). In terms of clinical use for direct patient care decisions, more stringent validation of these models across imaging modality and printing materials is necessary before it can be widely used to make crucial, real-time patient decisions (99,100). Cardiac structures are dynamic, and 3D models provide only one look at this changing structure. A more thorough understanding of the phase of the cardiac structure used for a model, and the properties of the materials used for printing will be necessary (99,101).
3D printing and modeling—conclusions
Despite its current limitations, 3D printing is an evolving technology within cardiology which will continue to expand as the technology and materials for printing evolve. The future of 3D printing is the potential for creation of custom implants or prosthesis, including heart valves and devices. At our center, Morrison et al., have already created such a device, an airway splint, for patients with tracheobronchomalacia (117). While a cardiac application is still in development, the ability to print a cardiac device, created to accommodate an individual patient’s anatomy is an exciting prospect and the epitome of personalized cardiac medicine.
Conclusions
Innovative techniques in cardiac catheterizations for patients with congenital heart disease are rooted in tremendous advances in both technology and imaging. These advances will continue to broaden our ability to treat patients using less-invasive techniques hopefully leading to both excellent immediate procedural success and also long-term improvement in patient outcomes compared to the state-of-the-art approaches of the recent past.
Acknowledgements
The authors would like to acknowledge Dr. Martin Bocks for providing his expertise on biodegradable technologies, Drs. Kanishka Ratnayaka and Elena Grant for providing their expertise and images of MRI-guided cardiac catheterization, and Dr. Michael Seckeler, for providing images of 3D printing.
Footnote
Conflicts of Interest: The authors have no conflicts of interest to declare.
References
- Bonhoeffer P, Boudjemline Y, Saliba Z, et al. Percutaneous replacement of pulmonary valve in a right-ventricle to pulmonary-artery prosthetic conduit with valve dysfunction. Lancet 2000;356:1403-5. [Crossref] [PubMed]
- McElhinney DB, Hellenbrand WE, Zahn EM, et al. Short- and medium-term outcomes after transcatheter pulmonary valve placement in the expanded multicenter US melody valve trial. Circulation 2010;122:507-16. [Crossref] [PubMed]
- Zahn EM, Hellenbrand WE, Lock JE, et al. Implantation of the melody transcatheter pulmonary valve in patients with a dysfunctional right ventricular outflow tract conduit early results from the u.s. Clinical trial. J Am Coll Cardiol 2009;54:1722-9. [Crossref] [PubMed]
- Armstrong AK, Balzer DT, Cabalka AK, et al. One-year follow-up of the Melody transcatheter pulmonary valve multicenter post-approval study. JACC Cardiovasc Interv 2014;7:1254-62. [Crossref] [PubMed]
- Butera G, Milanesi O, Spadoni I, et al. Melody transcatheter pulmonary valve implantation. results from the registry of the Italian Society of Pediatric Cardiology (SICP). Catheter Cardiovasc Interv 2013;81:310-6. [Crossref] [PubMed]
- Fraisse A, Aldebert P, Malekzadeh-Milani S, et al. Melody (R) transcatheter pulmonary valve implantation: results from a French registry. Arch Cardiovasc Dis 2014;107:607-14. [Crossref] [PubMed]
- Gillespie MJ, Dori Y, Harris MA, et al. Bilateral branch pulmonary artery melody valve implantation for treatment of complex right ventricular outflow tract dysfunction in a high-risk patient. Circ Cardiovasc Interv 2011;4:e21-3. [Crossref] [PubMed]
- Gillespie MJ, Rome JJ, Levi DS, et al. Melody valve implant within failed bioprosthetic valves in the pulmonary position: a multicenter experience. Circ Cardiovasc Interv 2012;5:862-70. [Crossref] [PubMed]
- Meadows JJ, Moore PM, Berman DP, et al. Use and performance of the Melody Transcatheter Pulmonary Valve in native and postsurgical, nonconduit right ventricular outflow tracts. Circ Cardiovasc Interv 2014;7:374-80. [Crossref] [PubMed]
- Shuto T, Kondo N, Dori Y, et al. Percutaneous transvenous Melody valve-in-ring procedure for mitral valve replacement. J Am Coll Cardiol 2011;58:2475-80. [Crossref] [PubMed]
- Garay F, Webb J, Hijazi ZM. Percutaneous replacement of pulmonary valve using the Edwards-Cribier percutaneous heart valve: first report in a human patient. Catheter Cardiovasc Interv 2006;67:659-62. [Crossref] [PubMed]
- Haas NA, Moysich A, Neudorf U, et al. Percutaneous implantation of the Edwards SAPIEN() pulmonic valve: initial results in the first 22 patients. Clin Res Cardiol 2013;102:119-28. [Crossref] [PubMed]
- Rockefeller T, Shahanavaz S, Zajarias A, et al. Transcatheter implantation of SAPIEN 3 valve in native right ventricular outflow tract for severe pulmonary regurgitation following tetralogy of fallot repair. Catheter Cardiovasc Interv 2016;88:E28-33. [Crossref] [PubMed]
- Suntharos P, Prieto LR. Percutaneous pulmonary valve implantation in the native right ventricular outflow tract using a 29-mm edwards SAPIEN 3 Valve. World J Pediatr Congenit Heart Surg 2017;8:639-42. [Crossref] [PubMed]
- Chatterjee A, Bajaj NS, McMahon WS, et al. Transcatheter pulmonary valve implantation: a comprehensive systematic review and meta-analyses of observational studies. J Am Heart Assoc 2017;6. [Crossref] [PubMed]
- Schievano S, Taylor AM, Capelli C, et al. First-in-man implantation of a novel percutaneous valve: a new approach to medical device development. EuroIntervention 2010;5:745-50. [Crossref] [PubMed]
- Bouzas B, Kilner PJ, Gatzoulis MA. Pulmonary regurgitation: not a benign lesion. Eur Heart J 2005;26:433-9. [Crossref] [PubMed]
- Frigiola A, Redington AN, Cullen S, et al. Pulmonary regurgitation is an important determinant of right ventricular contractile dysfunction in patients with surgically repaired tetralogy of Fallot. Circulation 2004;110:II153-7. [Crossref] [PubMed]
- Gatzoulis MA, Balaji S, Webber SA, et al. Risk factors for arrhythmia and sudden cardiac death late after repair of tetralogy of Fallot: a multicentre study. Lancet 2000;356:975-81. [Crossref] [PubMed]
- Knauth AL, Gauvreau K, Powell AJ, et al. Ventricular size and function assessed by cardiac MRI predict major adverse clinical outcomes late after tetralogy of Fallot repair. Heart 2008;94:211-6. [Crossref] [PubMed]
- Redington AN. Determinants and assessment of pulmonary regurgitation in tetralogy of Fallot: practice and pitfalls. Cardiol Clin 2006;24:631-9. [Crossref] [PubMed]
- Phillips AB, Nevin P, Shah A, et al. Development of a novel hybrid strategy for transcatheter pulmonary valve placement in patients following transannular patch repair of tetralogy of fallot. Catheter Cardiovasc Interv 2016;87:403-10. [Crossref] [PubMed]
- Sosnowski C, Matella T, Fogg L, et al. Hybrid pulmonary artery plication followed by transcatheter pulmonary valve replacement: Comparison with surgical PVR. Catheter Cardiovasc Interv 2016;88:804-10. [Crossref] [PubMed]
- Schoonbeek RC, Takebayashi S, Aoki C, et al. Implantation of the medtronic harmony transcatheter pulmonary valve improves right ventricular size and function in an ovine model of postoperative chronic pulmonary insufficiency. Circ Cardiovasc Interv 2016;9. [Crossref] [PubMed]
- Bergersen L, Benson LN, Gillespie MJ, et al. Harmony feasibility trial: acute and short-term outcomes with a self-expanding transcatheter pulmonary valve. JACC Cardiovasc Interv 2017;10:1763-73. [Crossref] [PubMed]
- Cao QL, Kenny D, Zhou D, et al. Early clinical experience with a novel self-expanding percutaneous stent-valve in the native right ventricular outflow tract. Catheter Cardiovasc Interv 2014;84:1131-7. [Crossref] [PubMed]
- Promphan W, Prachasilchai P, Siripornpitak S, et al. Percutaneous pulmonary valve implantation with the Venus P-valve: clinical experience and early results. Cardiol Young 2016;26:698-710. [Crossref] [PubMed]
- Garay F, Pan X, Zhang YJ, et al. Early experience with the Venus pvalve for percutaneous pulmonary valve implantation in native outflow tract. Neth Heart J 2017;25:76-81. [Crossref] [PubMed]
- Husain J, Praichasilchai P, Gilbert Y, et al. Early European experience with the Venus P-valve(R): filling the gap in percutaneous pulmonary valve implantation. EuroIntervention 2016;12:e643-51. [Crossref] [PubMed]
- Kim GB, Kwon BS, Lim HG. First in human experience of a new self-expandable percutaneous pulmonary valve implantation using knitted nitinol-wire and tri-leaflet porcine pericardial valve in the native right ventricular outflow tract. Catheter Cardiovasc Interv 2017;89:906-9. [Crossref] [PubMed]
- Bhati BS, Nandakumaran CP, Shatapathy P, et al. Closure of patent ductus arteriosus during open-heart surgery. Surgical experience with different techniques. J Thorac Cardiovasc Surg 1972;63:820-6. [PubMed]
- Akintuerk H, Michel-Behnke I, Valeske K, et al. Stenting of the arterial duct and banding of the pulmonary arteries: basis for combined Norwood stage I and II repair in hypoplastic left heart. Circulation 2002;105:1099-103. [Crossref] [PubMed]
- Gibbs JL, Wren C, Watterson KG, et al. Stenting of the arterial duct combined with banding of the pulmonary arteries and atrial septectomy or septostomy: a new approach to palliation for the hypoplastic left heart syndrome. Br Heart J 1993;69:551-5. [Crossref] [PubMed]
- Zampi JD, Hirsch JC, Goldstein BH, et al. Use of a pressure guidewire to assess pulmonary artery band adequacy in the hybrid stage I procedure for high-risk neonates with hypoplastic left heart syndrome and variants. Congenit Heart Dis 2013;8:149-58. [Crossref] [PubMed]
- Ashburn DA, McCrindle BW, Tchervenkov CI, et al. Outcomes after the Norwood operation in neonates with critical aortic stenosis or aortic valve atresia. J Thorac Cardiovasc Surg 2003;125:1070-82. [Crossref] [PubMed]
- Gaynor JW, Mahle WT, Cohen MI, et al. Risk factors for mortality after the Norwood procedure. Eur J Cardiothorac Surg 2002;22:82-9. [Crossref] [PubMed]
- Hehir DA, Dominguez TE, Ballweg JA, et al. Risk factors for interstage death after stage 1 reconstruction of hypoplastic left heart syndrome and variants. J Thorac Cardiovasc Surg 2008;136:94-9, 99.e1-3.
- Lim DS, Peeler BB, Matherne GP, et al. Risk-stratified approach to hybrid transcatheter-surgical palliation of hypoplastic left heart syndrome. Pediatr Cardiol 2006;27:91-5. [Crossref] [PubMed]
- Pizarro C, Derby CD, Baffa JM, et al. Improving the outcome of high-risk neonates with hypoplastic left heart syndrome: hybrid procedure or conventional surgical palliation? Eur J Cardiothorac Surg 2008;33:613-8. [Crossref] [PubMed]
- Rychik J, Rome JJ, Collins MH, et al. The hypoplastic left heart syndrome with intact atrial septum: atrial morphology, pulmonary vascular histopathology and outcome. J Am Coll Cardiol 1999;34:554-60. [Crossref] [PubMed]
- Stasik CN, Gelehrter S, Goldberg CS, et al. Current outcomes and risk factors for the Norwood procedure. J Thorac Cardiovasc Surg 2006;131:412-7. [Crossref] [PubMed]
- Wernovsky G, Kuijpers M, Van Rossem MC, et al. Postoperative course in the cardiac intensive care unit following the first stage of Norwood reconstruction. Cardiol Young 2007;17:652-65. [Crossref] [PubMed]
- Bacha EA, Daves S, Hardin J, et al. Single-ventricle palliation for high-risk neonates: the emergence of an alternative hybrid stage I strategy. J Thorac Cardiovasc Surg 2006;131:163-71.e2. [Crossref] [PubMed]
- Chen Q, Parry AJ. The current role of hybrid procedures in the stage 1 palliation of patients with hypoplastic left heart syndrome. Eur J Cardiothorac Surg 2009;36:77-83. [Crossref] [PubMed]
- Galantowicz M, Cheatham JP. Lessons learned from the development of a new hybrid strategy for the management of hypoplastic left heart syndrome. Pediatr Cardiol 2005;26:190-9. [Crossref]
- Galantowicz M, Cheatham JP, Phillips A, et al. Hybrid approach for hypoplastic left heart syndrome: intermediate results after the learning curve. Ann Thorac Surg 2008;85:2063-70; discussion 2070-1. [Crossref] [PubMed]
- Gutgesell HP, Lim DS. Hybrid palliation in hypoplastic left heart syndrome. Curr Opin Cardiol 2007;22:55-9. [Crossref] [PubMed]
- Venugopal PS, Luna KP, Anderson DR, et al. Hybrid procedure as an alternative to surgical palliation of high-risk infants with hypoplastic left heart syndrome and its variants. J Thorac Cardiovasc Surg 2010;139:1211-5. [Crossref] [PubMed]
- Karani KB, Zafar F, Morales DL, et al. Hybrid stage I palliation in a 1.1 kg, 28-week preterm neonate with posterior malalignment ventricular septal defect, left ventricular outflow tract obstruction, and coarctation of the aorta. World J Pediatr Congenit Heart Surg 2014;5:603-7. [Crossref] [PubMed]
- Uno Y, Masuoka A, Hotoda K, et al. Hybrid palliation for interrupted aortic arch with small aortic valve. World J Pediatr Congenit Heart Surg 2017;8:332-6. [Crossref] [PubMed]
- Hsia TY, Cosentino D, Corsini C, et al. Use of mathematical modeling to compare and predict hemodynamic effects between hybrid and surgical Norwood palliations for hypoplastic left heart syndrome. Circulation 2011;124:S204-10. [Crossref] [PubMed]
- Shimizu S, Kawada T, Une D, et al. Hybrid stage I palliation for hypoplastic left heart syndrome has no advantage on ventricular energetics: a theoretical analysis. Heart Vessels 2016;31:105-13. [Crossref] [PubMed]
- Zampi JD, Hirsch-Romano JC, Goldstein BH, et al. Hybrid approach for pulmonary atresia with intact ventricular septum: Early single center results and comparison to the standard surgical approach. Catheter Cardiovasc Interv 2014;83:753-61. [Crossref] [PubMed]
- Zhang H, Li SJ, Li YQ, et al. Hybrid procedure for the neonatal management of pulmonary atresia with intact ventricular septum. J Thorac Cardiovasc Surg 2007;133:1654-6. [Crossref] [PubMed]
- Agnoletti G, Piechaud JF, Bonhoeffer P, et al. Perforation of the atretic pulmonary valve. Long-term follow-up. J Am Coll Cardiol 2003;41:1399-403. [Crossref] [PubMed]
- Benson LN, Nykanen D, Collison A. Radiofrequency perforation in the treatment of congenital heart disease. Catheter Cardiovasc Interv 2002;56:72-82. [Crossref] [PubMed]
- Hasan BS, Bautista-Hernandez V, McElhinney DB, et al. Outcomes of transcatheter approach for initial treatment of pulmonary atresia with intact ventricular septum. Catheter Cardiovasc Interv 2013;81:111-8. [Crossref] [PubMed]
- Liava'a M, Brooks P, Konstantinov I, et al. Changing trends in the management of pulmonary atresia with intact ventricular septum: the Melbourne experience. Eur J Cardiothorac Surg 2011;40:1406-11. [PubMed]
- McLean KM, Pearl JM. Pulmonary atresia with intact ventricular septum: initial management. Ann Thorac Surg 2006;82:2214-9; discussion 2219-20. [Crossref] [PubMed]
- Ovaert C, Qureshi SA, Rosenthal E, et al. Growth of the right ventricle after successful transcatheter pulmonary valvotomy in neonates and infants with pulmonary atresia and intact ventricular septum. J Thorac Cardiovasc Surg 1998;115:1055-62. [Crossref] [PubMed]
- Qureshi SA. Catheterization in neonates with pulmonary atresia with intact ventricular septum. Catheter Cardiovasc Interv 2006;67:924-31. [Crossref] [PubMed]
- Zampi JD, Loccoh E, Armstrong AK, et al. Twenty years of experience with intraoperative pulmonary artery stenting. Catheter Cardiovasc Interv 2017;90:398-406. [Crossref] [PubMed]
- Levi DS, Cheng AL. Biodegradable Implants. In: Da Cruz EM, Ivy D, Jaggers J, editors. Pediatric and Congenital Cardiology, Cardiac Surgery and Intensive Care. London: Springer; 2014:1219-35.
- Solorio LD, Bocks ML, Hollister SJ. Tailoring the physicochemical and shape memory properties of the biodegradable polymer poly(glycerol dodecanoate) via curing conditions. J Biomed Mater Res A 2017;105:1618-23. [Crossref] [PubMed]
- Bowen PK, Guillory RJ 2nd, Shearier ER, et al. Metallic zinc exhibits optimal biocompatibility for bioabsorbable endovascular stents. Mater Sci Eng C Mater Biol Appl 2015;56:467-72. [Crossref] [PubMed]
- Boe BA, Zampi JD, Schumacher KR, et al. The use and outcomes of small, medium and large premounted stents in pediatric and congenital heart disease. Pediatr Cardiol 2016;37:1525-33. [Crossref] [PubMed]
- Morgan G, Lee KJ, Chaturvedi R, et al. A biodegradable device (BioSTAR) for atrial septal defect closure in children. Catheter Cardiovasc Interv 2010;76:241-5. [Crossref] [PubMed]
- Rizik DG, Hermiller JB, Kereiakes DJ. The ABSORB bioresorbable vascular scaffold: A novel, fully resorbable drug-eluting stent: Current concepts and overview of clinical evidence. Catheter Cardiovasc Interv 2015;86:664-77. [Crossref] [PubMed]
- Gogas BD, King SB 3rd, Samady H. Bioresorbable polymeric scaffolds for coronary revascularization: Lessons learnt from ABSORB III, ABSORB China, and ABSORB Japan. Glob Cardiol Sci Pract 2015;2015:62. [Crossref] [PubMed]
- Kleinerman RA. Cancer risks following diagnostic and therapeutic radiation exposure in children. Pediatr Radiol 2006;36 Suppl 2:121-5. [Crossref] [PubMed]
- Johnson JN, Hornik CP, Li JS, et al. Cumulative radiation exposure and cancer risk estimation in children with heart disease. Circulation 2014;130:161-7. [Crossref] [PubMed]
- Beels L, Bacher K, De Wolf D, et al. gamma-H2AX foci as a biomarker for patient X-ray exposure in pediatric cardiac catheterization: are we underestimating radiation risks? Circulation 2009;120:1903-9. [Crossref] [PubMed]
- Ait-Ali L, Andreassi MG, Foffa I, et al. Cumulative patient effective dose and acute radiation-induced chromosomal DNA damage in children with congenital heart disease. Heart 2010;96:269-74. [Crossref] [PubMed]
- Razavi R, Hill DL, Keevil SF, et al. Cardiac catheterisation guided by MRI in children and adults with congenital heart disease. Lancet 2003;362:1877-82. [Crossref] [PubMed]
- Krueger JJ, Ewert P, Yilmaz S, et al. Magnetic resonance imaging-guided balloon angioplasty of coarctation of the aorta: a pilot study. Circulation 2006;113:1093-100. [Crossref] [PubMed]
- Ratnayaka K, Faranesh AZ, Hansen MS, et al. Real-time MRI-guided right heart catheterization in adults using passive catheters. Eur Heart J 2013;34:380-9. [Crossref] [PubMed]
- Tzifa A, Krombach GA, Kramer N, et al. Magnetic resonance-guided cardiac interventions using magnetic resonance-compatible devices: a preclinical study and first-in-man congenital interventions. Circ Cardiovasc Interv 2010;3:585-92. [Crossref] [PubMed]
- Ratnayaka K, Kanter JP, Faranesh AZ, et al. Radiation-free CMR diagnostic heart catheterization in children. J Cardiovasc Magn Reson 2017;19:65. [Crossref] [PubMed]
- Ratnayaka K, Rogers T, Schenke WH, et al. Magnetic resonance imaging-guided transcatheter cavopulmonary shunt. JACC Cardiovasc Interv 2016;9:959-70. [Crossref] [PubMed]
- Benndorf G, Klucznik RP, Strother CM. Images in cardiovascular medicine. Angiographic computed tomography for imaging of underdeployed intracranial stent. Circulation 2006;114:e499-500. [Crossref] [PubMed]
- Heran NS, Song JK, Namba K, et al. The utility of DynaCT in neuroendovascular procedures. AJNR Am J Neuroradiol 2006;27:330-2. [PubMed]
- Borik S, Volodina S, Chaturvedi R, et al. Three-dimensional rotational angiography in the assessment of vascular and airway compression in children after a cavopulmonary anastomosis. Pediatr Cardiol 2015;36:1083-9. [Crossref] [PubMed]
- Truong UT, Fagan TE, Deterding R, et al. Use of rotational angiography in assessing relationship of the airway to vasculature during cardiac catheterization. Catheter Cardiovasc Interv 2015;86:1068-77. [Crossref] [PubMed]
- Glatz AC, Zhu X, Gillespie MJ, et al. Use of angiographic CT imaging in the cardiac catheterization laboratory for congenital heart disease. JACC Cardiovasc Imaging 2010;3:1149-57. [Crossref] [PubMed]
- Loomba RS, Rios R, Buelow M, et al. Comparison of contrast volume, radiation dose, fluoroscopy time, and procedure time in previously published studies of rotational versus conventional coronary angiography. Am J Cardiol 2015;116:43-9. [Crossref] [PubMed]
- Haddad L, Waller BR, Johnson J, et al. Radiation protocol for three-dimensional rotational angiography to limit procedural radiation exposure in the pediatric cardiac catheterization lab. Congenit Heart Dis 2016;11:637-46. [Crossref] [PubMed]
- Aldoss O, Fonseca BM, Truong UT, et al. Diagnostic utility of three-dimensional rotational angiography in congenital cardiac catheterization. Pediatr Cardiol 2016;37:1211-21. [Crossref] [PubMed]
- Berman DP, Khan DM, Gutierrez Y, et al. The use of three-dimensional rotational angiography to assess the pulmonary circulation following cavo-pulmonary connection in patients with single ventricle. Catheter Cardiovasc Interv 2012;80:922-30. [Crossref] [PubMed]
- Dori Y, Sarmiento M, Glatz AC, et al. X-Ray magnetic resonance fusion to internal markers and utility in congenital heart disease catheterization. Circ Cardiovasc Imaging 2011;4:415-24. [Crossref] [PubMed]
- Gutiérrez LF, Silva R, Ozturk C, et al. Technology preview: X-ray fused with magnetic resonance during invasive cardiovascular procedures. Catheter Cardiovasc Interv 2007;70:773-82. [Crossref] [PubMed]
- Abu Hazeem AA, Dori Y, Whitehead KK, et al. X-ray magnetic resonance fusion modality may reduce radiation exposure and contrast dose in diagnostic cardiac catheterization of congenital heart disease. Catheter Cardiovasc Interv 2014;84:795-800. [Crossref] [PubMed]
- Glöckler M, Halbfabeta J, Koch A, et al. Multimodality 3D-roadmap for cardiovascular interventions in congenital heart disease--a single-center, retrospective analysis of 78 cases. Catheter Cardiovasc Interv 2013;82:436-42. [Crossref] [PubMed]
- Thaden JJ, Sanon S, Geske JB, et al. Echocardiographic and fluoroscopic fusion imaging for procedural guidance: an overview and early clinical experience. J Am Soc Echocardiogr 2016;29:503-12. [Crossref] [PubMed]
- Sündermann SH, Biaggi P, Grünenfelder J, et al. Safety and feasibility of novel technology fusing echocardiography and fluoroscopy images during MitraClip interventions. EuroIntervention 2014;9:1210-6. [Crossref] [PubMed]
- Gafoor S, Schulz P, Heuer L, et al. Use of echonavigator, a novel echocardiography-fluoroscopy overlay system, for transseptal puncture and left atrial appendage occlusion. J Interv Cardiol 2015;28:215-7. [Crossref] [PubMed]
- Balzer J, Zeus T, Hellhammer K, et al. Initial clinical experience using the EchoNavigator((R))-system during structural heart disease interventions. World J Cardiol 2015;7:562-70. [Crossref] [PubMed]
- Jone PN, Ross MM, Bracken JA, et al. Feasibility and safety of using a fused echocardiography/fluoroscopy imaging system in patients with congenital heart disease. J Am Soc Echocardiogr 2016;29:513-21. [Crossref] [PubMed]
- Fagan TE, Truong UT, Jone PN, et al. Multimodality 3-Dimensional image integration for congenital cardiac catheterization. Methodist Debakey Cardiovasc J 2014;10:68-76. [Crossref] [PubMed]
- Vukicevic M, Mosadegh B, Min JK, et al. Cardiac 3D Printing and its Future Directions. JACC Cardiovasc Imaging 2017;10:171-84. [Crossref] [PubMed]
- Abudayyeh I, Gordon B, Ansari MM, et al. A practical guide to cardiovascular 3D printing in clinical practice: Overview and examples. J Interv Cardiol 2017. [Epub ahead of print]. [Crossref] [PubMed]
- Cantinotti M, Valverde I, Kutty S. Three-dimensional printed models in congenital heart disease. Int J Cardiovasc Imaging 2017;33:137-44. [Crossref] [PubMed]
- Monney P, Piccini D, Rutz T, et al. Single centre experience of the application of self navigated 3D whole heart cardiovascular magnetic resonance for the assessment of cardiac anatomy in congenital heart disease. J Cardiovasc Magn Reson 2015;17:55. [Crossref] [PubMed]
- Gilon D, Cape EG, Handschumacher MD, et al. Effect of three-dimensional valve shape on the hemodynamics of aortic stenosis: three-dimensional echocardiographic stereolithography and patient studies. J Am Coll Cardiol 2002;40:1479-86. [Crossref] [PubMed]
- Binder TM, Moertl D, Mundigler G, et al. Stereolithographic biomodeling to create tangible hard copies of cardiac structures from echocardiographic data: in vitro and in vivo validation. J Am Coll Cardiol 2000;35:230-7. [Crossref] [PubMed]
- Olivieri LJ, Krieger A, Loke YH, et al. Three-dimensional printing of intracardiac defects from three-dimensional echocardiographic images: feasibility and relative accuracy. J Am Soc Echocardiogr 2015;28:392-7. [Crossref] [PubMed]
- Gosnell J, Pietila T, Samuel BP, et al. Integration of computed tomography and three-dimensional echocardiography for hybrid three-dimensional printing in congenital heart disease. J Digit Imaging 2016;29:665-9. [Crossref] [PubMed]
- Mahmood F, Owais K, Taylor C, et al. Three-dimensional printing of mitral valve using echocardiographic data. JACC Cardiovasc Imaging 2015;8:227-9. [Crossref] [PubMed]
- Vranicar M, Gregory W, Douglas WI, et al. The use of stereolithographic hand held models for evaluation of congenital anomalies of the great arteries. Stud Health Technol Inform 2008;132:538-43. [PubMed]
- Schievano S, Migliavacca F, Coats L, et al. Percutaneous pulmonary valve implantation based on rapid prototyping of right ventricular outflow tract and pulmonary trunk from MR data. Radiology 2007;242:490-7. [Crossref] [PubMed]
- Ngan EM, Rebeyka IM, Ross DB, et al. The rapid prototyping of anatomic models in pulmonary atresia. J Thorac Cardiovasc Surg 2006;132:264-9. [Crossref] [PubMed]
- Sodian R, Weber S, Markert M, et al. Stereolithographic models for surgical planning in congenital heart surgery. Ann Thorac Surg 2007;83:1854-7. [Crossref] [PubMed]
- Farooqi KM, Nielsen JC, Uppu SC, et al. Use of 3-dimensional printing to demonstrate complex intracardiac relationships in double-outlet right ventricle for surgical planning. Circ Cardiovasc Imaging 2015;8. [Crossref] [PubMed]
- Velasco Forte MN, Byrne N, Valverde I, et al. Interventional correction of sinus venosus atrial septal defect and partial anomalous pulmonary venous drainage: procedural planning using 3D Printed Models. JACC Cardiovasc Imaging 2018;11:275-8. [Crossref] [PubMed]
- Grant EK, Olivieri LJ. The Role of 3-D Heart Models in Planning and Executing Interventional Procedures. Can J Cardiol 2017;33:1074-81. [Crossref] [PubMed]
- Little SH, Vukicevic M, Avenatti E, et al. 3D printed modeling for patient-specific mitral valve intervention: repair with a clip and a plug. JACC Cardiovasc Interv 2016;9:973-5. [Crossref] [PubMed]
- Biglino G, Verschueren P, Zegels R, et al. Rapid prototyping compliant arterial phantoms for in-vitro studies and device testing. J Cardiovasc Magn Reson 2013;15:2. [Crossref] [PubMed]
- Morrison RJ, Hollister SJ, Niedner MF, et al. Mitigation of tracheobronchomalacia with 3D-printed personalized medical devices in pediatric patients. Sci Transl Med 2015;7:285ra64. [Crossref] [PubMed]