New concepts in the pathogenesis of hydrocephalus
Introduction
Hydrocephalus is a central nervous system disorder characterized by excessive accumulation of cerebrospinal fluid (CSF) in the ventricles of the brain. Although this disorder is common in childhood, hydrocephalus can occur at any age and results in significant cognitive and physical handicap. A wide variety of disorders including brain tumors, strokes, infections and hemorrhage affect the central nervous system and can result in hydrocephalus. Hydrocephalus results in significant health burden in the developing countries; in the United States it is estimated to cost $2 billion annually (1). Treatment options for relieving hydrocephalus are primarily surgical by insertion of a diverting shunt or by performing an endoscopic third ventriculostomy. Both methods have drawbacks: shunts fail often despite significant improvements in technology (2) and the results of third ventriculostomy are not consistent and difficult to predict. Given these risks, the patient is guaranteed to a lifetime of monitoring with diagnostic tests and more surgery.
Improvement of the current state of diagnosis and treatment of hydrocephalus involves a better understanding of the pathophysiology of hydrocephalus. This review will begin by examining the circulation theory, additional data outside of the scope of circulation theory, data supporting the role of osmotic gradients and suggest a potential construct to explain how hydrocephalus occurs.
The concept of circulation theory
Circulation theory states that CSF is actively produced from choroid plexuses in the ventricles and flows from the lateral ventricles through the foramen of Monro, the third ventricle, aqueduct, the fourth ventricle, and then through the foramen of Luschka and Magendie into the subarachnoid space (SAS) where it is passively absorbed into cranial venous sinuses into the blood (3) (Figure 1). Circulation theory was proposed a century ago (4,5) and is based on three key premises (1) the active formation or secretion of CSF (2), the passive absorption of CSF and (3) unidirectional flow of CSF from the place of formation to the place of absorption (3). These premises led to the description of CSF circulation as the third circulation (after blood and lymphatic circulations) (5-7).
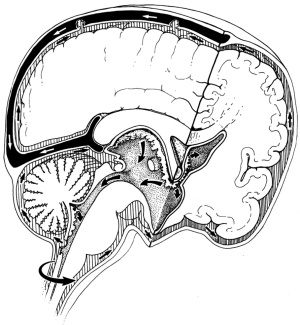
CSF formation
It is generally assumed that the main production sites of CSF are the choroid plexuses (contributing 70-80%) where the filtration across the endothelial wall of the capillaries and the secretion through choroidal epithelium occur. The remaining 20-30% of CSF production arises as a bulk flow of the interstitial fluid (also known as the extrachoroidal source) likely produced by the ependyma (8-11). Formation of CSF is assumed to be an active process independent of intracranial pressure and therefore an obstruction of the CSF pathways will lead to hydrocephalus (12,13).
CSF circulation
It is believed that flow of CSF results from the pulsatile pumping action of the choroid plexuses that is generated by the filling and draining of choroid plexuses (14). Each pulse within the choroid plexus will force the CSF out of the ventricles into the SAS. Additionally, there is some flow of the CSF into the spinal SAS, likely with a lower intensity (15).
CSF absorption
Arachnoid villi inside the dural venous sinuses have been generally thought to be the main site of CSF absorption. It is believed that CSF is passively absorbed from the cranial SAS to the cranial venous blood by means of a hydrostatic gradient (16). Initially the villi on the arachnoid granulation were described as an open tubular system projecting from the granulation tissue into the venous sinus (17,18). The ultrastructural studies have not been consistent in support of these pressure sensitive openings through the arachnoid villi (19,20).
Therefore, according to circulation theory, hydrocephalus is a result of a discrepancy between the amount of CSF produced and the amount of CSF absorbed. Any condition that results in the blockage of the normal flow of CSF or its absorption will result in hydrocephalus. Recently Rekate proposed a new definition of hydrocephalus that illustrates these concepts: ‘‘Hydrocephalus is an active distension of the ventricular system of the brain resulting from the inadequate passage of CSF from its point of production within the cerebral ventricles to its point of absorption into the systemic circulation.’’ (21). Hydrocephalus was classified into six different types depending on the site of obstruction: foramen of Monro, aqueduct of Sylvius, basal cisterns, arachnoid granulations, venous outflow and overproduction by choroid plexus papilloma (21).
What is the supporting data for circulation theory?
The rate of formation of CSF has been demonstrated as a pressure dependent process and the rate decreases as the CSF pressure is increased (22-24). CSF is also formed in the cranial and spinal SAS (extrachoroidal formation) (25-27). The theory stating that the choroid plexus is the main source of CSF formation is further challenged by the observation that volume and composition of CSF does not change when choroid plexuses has been removed (28).
Dandy and Blackfan [1913] were the first to induce experimental hydrocephalus by obstructing the aqueduct in a dog using a cotton pledget in a capsule (4). The ventricles proximal to the occlusion became dilated; however, the fourth ventricle did not enlarge. Following this, many models of hydrocephalus have been used to study the impaired circulation of CSF and assess the efficacy of therapeutic measures. Obstructive agents include irritative agents such as kaolin, India ink, pantopaque, silastic, silicone oil, blood, cotton etc. into the CSF space (29-33).
All the models tested had pathophysiological mechanisms such as inflammation and brain damage (resulting from the introduction of a foreign material) in addition to obstruction of the CSF pathways. This compounds the analysis of causation of hydrocephalus (3). Aqueductal stenosis has been associated with hydrocephalus and considered to be causative. Aqueductal stenosis has been shown to follow the development of hydrocephalus in both animal models (34,35) and in humans (36,37). However, there is evidence to show that aqueductal stenosis may in fact be the result of hydrocephalus, instead of the cause of hydrocephalus. First, aqueductal obstruction without induced inflammation did not result in dilation of ventricles or even an increase in pressure compared to control animals (38). Secondly, there is no evidence to pinpoint the exact routes taken by the CSF from the choroid plexus in the lateral ventricles through the aqueduct. In fact, Fenstermacher showed that 14Csucrose, when injected into the lateral ventricles, moves into the third ventricle and onto the basal cisterns through the roof of the third ventricle before going into the aqueduct (39). Moreover, even in the presence of complete blockage of the CSF pathways and the aqueducts, nearly a quarter of animal models tested did not get hydrocephalus in an experimental model using kaolin (40).
If obstructions in the CSF pathways drive the development of hydrocephalus, there should be a corresponding change in the transmantle pressure gradients. Transmantle pressure gradient is the difference between the intraventricular pressure and the pressure in the SASs. This gradient has been hypothesized to be the driving force of ventricular dilatation. Such a gradient has not been demonstrated in experimental animals (41) or in humans (42).
It was shown by Oresković et al. that CSF formation and absorption are in balance at physiological ICPs within the isolated lateral ventricles (24). This implies that CSF is significantly absorbed inside the ventricles and not just the venous sinuses (43-45). CSF is also absorbed from the SAS to the lymphatics and this is preserved across the different species of mammals (46-51).
As demonstrated, the CSF circulation theory for the development of hydrocephalus is belied by various clinical observations and several experimental findings (52-57).
Why osmotic gradients have a role in the pathogenesis?
One of the fundamental assumptions of circulation theory is that the brain parenchyma is impermeable to CSF, and is therefore incapable of absorbing the CSF accumulating within the ventricles. However, the brain parenchyma is permeable to water (58). The molecular basis of this permeability involves specific ion channels that permit water movement with ions as well as aquaporin channels, which permit the free movement of water without changing the ionic environment (59). Aquaporin channels are membrane proteins that have an ion trap and allow movement of water without allowing movement of ions. Aquaporin 4 (AQP4) channels are found in the ependymal cells lining the lateral ventricles, and on the end feet of astrocytes. These astrocytes, in particular, contact microvessels in the periventricular white matter and the subpial region of the cerebral cortex (60). The distribution of AQP4 within the brain suggests that the aqueous content of CSF may be increased or decreased as water moves through the brain parenchyma between the ventricles and vascular system.
Since brain tissue is permeable to water, it implies that changes in the concentration of the osmotically active chemicals in the ventricular/interstitial fluid results in a change in the local osmotic gradient. Any osmotic gradient between the ventricular or interstitial CSF and the blood should be equilibrated with transport of water between the two compartments. Therefore, we will examine the role of osmotic gradients in the pathogenesis of hydrocephalus. It is well known clinically that osmotic gradients play a role in the brain tissues (excluding the ventricular space) in normal and abnormal states. For example, in brain edema, osmotic diuretics like mannitol are used intravenously to draw water away from the extracellular space of the brain. Brain swelling can result in conditions where there is hyponatremia that permits water movement into the tissues of the brain resulting in brain edema (61).
Proteins are large macromolecules that are not diffusible through the blood brain barrier and therefore contribute to larger osmotic content in any fluid compartment. Higher protein content in a fluid compartment will change the osmotic gradient in favor of water transport into the compartment from the blood. Clinically, high protein levels in the CSF have been detected in hydrocephalus irrespective of radiologic obstruction to the CSF pathways. Higher levels of thrombopoietin (62), ferritin (63), chondroitin sulfate proteoglycan (64) transforming growth factor beta 1 (65,66), transforming growth factor beta 2 (65), and vascular endothelial growth factor (67) have been found in ventricular CSF in patients with hydrocephalus resulting from intraventricular hemorrhage. Nerve growth factor (68) and S-100 protein (69,70) are found to be elevated in patients with hydrocephalus without intraventricular hemorrhage. In addition, elevated proteins have been found in ventricular CSF in hydrocephalus resulting from intracranial schwannomas (71), a few cases of spinal schwannomas (72,73), and in about 4% of patients with Guillain-Barre’ syndrome (74,75). In a review of potential biomarkers for chronic hydrocephalus, Tarnaris et al. concluded that tumor necrosis factor, tau protein, lactate, sulfatide and neurofilament triple protein are elevated in chronic hydrocephalus to make them the most promising CSF markers (76). In addition to proteins, increased levels of lactate (76,77) and lactic dehydrogenase (LDH) (78) have been found in hydrocephalus. Increased levels of ions (calcium, magnesium and phosphate) found in congenital hydrocephalus correlated with elevated protein levels (79). Several of these changes in the composition of CSF in hydrocephalus were summarized in a review article by Del Bigio (80).
The importance of the role played by excess macromolecules in the ventricles in hydrocephalus is strengthened by the relief of hydrocephalus in situations that decrease the amount of macromolecules in the CSF. Decreasing levels of protein and blood products with removal of CSF through an Ommaya ventricular reservoir is associated with resolution of hydrocephalus in about 17% of neonates with intraventricular hemorrhage (81,82). The elimination of blood and blood products decreases the incidence of hydrocephalus due to aneurysmal subarachnoid hemorrhage (83). The elimination of macromolecules results in the relief of hydrocephalus, which may be explained by the decrease in the osmotic load and the reduction of the macromolecules’ biological effect on the brain and CSF secretion.
In addition to the clinical evidence, experimental evidence also suggests that osmolality plays a role in the genesis of hydrocephalus. Wald et al. found that increasing the ventricular fluid osmolality in a perfusate increased the volume of CSF produced (84) and increasing the serum osmolality decreased CSF production in normal cats (85). These experiments led the authors to conclude that CSF production is influenced by the osmotic gradient between the serum and the ventricular CSF. In other experiments, infusion of proteins [FGF-2 (86), thrombin (87)] into the lateral ventricles of experimental animals caused dilatation of the ventricles. Our laboratory research has demonstrated that altering the CSF osmotic gradient by infusing hyperosmolar dextran into the cerebral ventricles results in hydrocephalus (88) (Figure 2). Further, the severity of hydrocephalus is proportional to the increase in the osmotic load in the ventricles (89). Other investigators have also confirmed the effect of osmotic gradients in the development of hydrocephalus (90-92). These results suggest that water transport into the ventricles is secondary to the osmotic load or the amount of macromolecules in the ventricles.
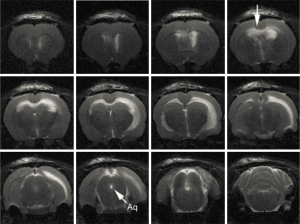
Further support for the concept of osmotic gradients resulting in hydrocephalus comes from experiments focusing on the development of ventricles in embryos. It is well known that brain ventricles are a highly conserved system of cavities that form early during brain morphogenesis in vertebrates and are required for normal brain function (93). The neural tube is a single cell layer tube that is permeable to water. Expansion of this tube involves changes in the osmolality of the neural tube fluid. Alonso et al., during an investigation of the underlying mechanism of neural tube expansion in chick embryos found that increasing the neural tube fluid osmolality resulted in hydrocephalus (93). In a series of elegantly done experiments, Lowery and Sive were able to show that initial ventricle expansion occurs independently of circulation and is related to cellular proliferation in zebrafish embryos (90). Although onset of circulation contributed to continued expansion of the ventricles, ventricular expansion occurred in silent heart mutant zebrafish embryos that do not have a beating heart (90).
Paravascular and lymphatic pathways involved in macromolecular clearance
If osmotic gradients caused by the presence of macromolecules in the ventricles result in hydrocephalus, then it is important to understand how these macromolecules are cleared from the ventricles. We found that macromolecules infused into the ventricles are cleared through the brain parenchyma along the perivascular spaces and along the cribriform plate into the nose (paper being submitted for publication). These findings, that the macromolecules infused into the ventricles or intrathecal spaces are distributed in the paravascular pathways, are consistent with the results of other authors (94,95). These pathways are also termed as the glymphatic pathways or system. Rennels et al. infused horseradish peroxidase (HRP) into the lateral ventricles and found that there is a rapid paravascular influx of HRP-a faster influx than can be expected from diffusion (95). They found that decreasing the arterial pulsations decreased the movement of HRP through these spaces (95). The same group found that this transport of HRP through the paravascular pathways was limited by focal cerebral edema (96). Zhang et al. (97) injected india ink into the cerebral white and gray matter and into the SAS and found that the tracer is transported along specific paravascular pathways. Further, subarachnoid injection resulted in transport of the tracer along the paravascular pathways as well as through the lymphatics in the nose. The observation that macromolecules or tracers in the ventricles are transported along paravascular pathways has been confirmed by observing the tracer movement using confocal microscopy (98) and MR imaging (99) recently.
Where do the macromolecules transported through the paravascular pathways go? Zhang et al. found that the particulate matter that is injected is rapidly and efficiently ingested by perivascular cells (97). The authors summarized these findings in a review article and highlighted both the paravascular and the nasal lymphatic pathways and their immunological significance (100). The authors proposed that this absorption of the macromolecules by immunologically competent cells as an explanation for immune mediated CNS disorders. The exact mechanism of macromolecular clearance out of the brain is uncertain.
Venous system plays an important role
Williams has extensively reviewed the significant role played by the venous system in the pathogenesis of hydrocephalus (101). There are many disease processes that can lead to a state of CNS venous insufficiency by causing an increase in arterial supply, filtration of fluid into the parenchyma or venous pressure. An incomplete list includes CNS infection, hypoxia with altitude, carbon monoxide poisoning, water intoxication, renal impairment, obesity, and anemia (102). Cardiac failure is an infrequent cause of hydrocephalus (103). Hydrocephalus can result from the compression of the venous channels by single suture or multiple suture craniosynostosis (104). Venous hypertension from unruptured arteriovenous malformations can result in hydrocephalus (105-107). Given the above clinical evidence of the relationship between venous insufficiency or venous hypertension and hydrocephalus, it is appropriate to state that venous drainage is important in the pathophysiology of hydrocephalus.
Summary
Hydrocephalus is a complex condition resulting from a wide variety of different disorders. Circulation theory although widely accepted to be representative of how hydrocephalus develops does not have adequate proof either in clinical situations or in experimental setting. There is significant evidence that osmotic gradients are responsible for water content of the ventricles of the brain just as they are in other water permeable organs in the body. Any disorder that results in excess macromolecules in the ventricular fluid will change the osmotic gradient and result in hydrocephalus. Alternatively, we can view hydrocephalus as a disorder of macromolecular clearance (Figure 3). Evidence points to a paravascular or lymphatic clearance of these macromolecules out of the ventricles and the brain into the venous system. Although there are clearly some gaps in this pathophysiological construct as well, this seems to have considerably more support.
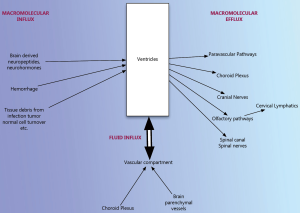
Acknowledgements
None.
Footnote
Conflicts of Interest: The authors have no conflicts of interest to declare.
References
- Simon TD, Riva-Cambrin J, Srivastava R, et al. Hospital care for children with hydrocephalus in the United States: utilization, charges, comorbidities, and deaths. J Neurosurg Pediatr 2008;1:131-7. [PubMed]
- Warf BC. Comparison of 1-year outcomes for the Chhabra and Codman-Hakim Micro Precision shunt systems in Uganda. a prospective study in 195 children. J Neurosurg 2005;102:358-62. [PubMed]
- Orešković D, Klarica M. Development of hydrocephalus and classical hypothesis of cerebrospinal fluid hydrodynamics: Facts and illusions. Prog Neurobiol 2011;94:238-58. [PubMed]
- Dandy WE, Blackfan KD. An experimental and clinical study of internal hydrocephalus. J Am Med Assoc 1913;61:2216-7.
- Cushing H. Studies on the cerebrospinal fluid. J Med Res 1914;31:1-19. [PubMed]
- Taketomo T, Saito A. Experimental studies on cerebrospinal fluid flow. Neurology 1965;15:578-86. [PubMed]
- Milhorat TH. The third circulation revisited. J Neurosurg 1975;42:628-45. [PubMed]
- O’Connell JE. Cerebrospinal fluid mechanics. Proc R Soc Med 1970;63:507-18. [PubMed]
- Milhorat TH. eds. Hydrocephalus and the Cerebrospinal fluid. Baltimore: Williams & Wilkins, 1972.
- Pollay M. Formation of cerebrospinal fluid. J Neurosurg 1975;42:665-73. [PubMed]
- McComb JG. Recent research into nature of cerebrospinal fluid formation and absorption. J Neurosurg 1983;59:369-83. [PubMed]
- Davson H, Welch K, Segal MB. eds. Physiology and pathophysiology of the cerebrospinal fluid. Edinburgh: Churchill-Livingstone, 1987.
- Pollay M, Stevens A, Roberts PA. Alteration in choroid plexus blood flow and cerebrospinal fluid formation by increased ventricular pressure. In: Wood JH. eds. Neurobiology of Cerebrospinal Fluid. New York: Plenum Press, 1983:687-95.
- Bering EA Jr. Choroid plexus and arterial pulsations of the cerebrospinal fluid. Demonstration of the choroid plexuses as a cerebrospinal fluid pump. AMA Arch Neurol Psychiatry 1955;73:165-72. [PubMed]
- Di Chiro G. Observations on the circulation of the cerebrospinal fluid. Acta Radiol Diagn (Stockh) 1966;5:988-1002. [PubMed]
- Weed LH. Forces concerned in the absorption of the cerebrospinal fluid. Am J Physiol 1935;114:40-5.
- Welch K, Friedman V. The cerebrospinal fluid valves. Brain 1960;83:454-69. [PubMed]
- Welch K, Pollay M. Perfusion of particles through arachnoid villi of the monkey. Am J Physiol 1961;201:651-4. [PubMed]
- Shabo AL, Maxwell DS. The morphology of the arachnoid villi: a light and electron microscopic study in the monkey. J Neurosurg 1968;29:451-63.
- Tripathi BJ, Tripathi R. Vacuolar transcellular channels as a drainage pathways for cerebrospinal fluid. J Physiol (Lond) 1974;239:195-206. [PubMed]
- Rekate HL. The definition and classification of hydrocephalus: a personal recommendation to stimulate debate. Cerebrospinal Fluid Res 2008;5:2. [PubMed]
- Flexner IB, Winters H. The rate of formation of cerebrospinal fluid in etherized cats. Am J Physiol 1932;101:697-710.
- Hochwald GM, Sahar A. Effect of spinal fluid pressure on cerebrospinal fluid formation. Exp Neurol 1971;32:30-40. [PubMed]
- Oresković D, Whitton PS, Lupret V. Effect of intracranial pressure on cerebrospinal fluid formation in isolated brain ventricles. Neuroscience 1991;41:773-7. [PubMed]
- Sato O, Bering EA. Extraventricular formation of cerebrospinal fluid. No To Shinkei 1967;19:883-5. [PubMed]
- Sato O, Asai T, Amano Y, et al. Formation of cerebrospinal fluid in spinal subarachnoidal space. Nature 1971;233:129-30. [PubMed]
- Sato O, Asai T, Amano Y, et al. Extraventricular origin of cerebrospinal fluid; formation rate qualitatively measured in the subarachnoid space of dogs. J Neurosurg 1972;36:276-82. [PubMed]
- Milhorat TH. Choroid plexus and cerebrospinal fluid production. Science 1969;166:1514-6. [PubMed]
- Bering EA Jr, Sato O. Hydrocephalus. changes in formation and absorption of cerebrospinal fluid within the cerebral ventricles. J Neurosurg 1963;20:1050-63. [PubMed]
- Dandy WE. Experimental hydrocephalus. Ann Surg 1919;70:129-42. [PubMed]
- Edwards MS, Harrison MR, Halks-Miller M, et al. Kaolin induced congenital hydrocephalus in fetal lambs and rhesus monkeys. J Neurosurg 1984;60:115-22. [PubMed]
- Hochwald GM. Animal models of hydrocephalus; recent developments Proc. Soc Exp Biol Med 1985;178:1-11. [PubMed]
- Milhorat TH, Clark RG, Hammock MK. Experimental hydrocephalus Part 2. Gross pathological findings in acute and subacute obstructive hydrocephalus in the dog and monkey. J Neurosurg 1970;32:390-9. [PubMed]
- Davis LE. Communicating hydrocephalus in newborn hamsters and cats following vaccine virus infection. J Neurosurg 1981;54:767-72. [PubMed]
- Masters C, Alpers M, Kakulas B. Pathogenesis of reovirus type 1 hydrocephalus in mice. Arch Neurol 1977;34:18-28. [PubMed]
- Foltz EL, Shurtleff DB. Conversion of communicating hydrocephalus to stenosis or occlusion of the aqueduct during ventricular shunt. J Neurosurg 1966;24:520-9. [PubMed]
- Williams B. Is aqueduct stenosis a result of hydrocephalus? Brain 1973;96:399-412. [PubMed]
- Klarica M, Oreskovic D, Bozic B, et al. New experimental model of acute aqueductal blockade in cats; effects on cerebrospinal fluid pressure and the size of brain ventricles. Neuroscience 2009;158:1397-405. [PubMed]
- Fenstermacher JD, Ghersi-Egea JF, Finnegan W, et al. The rapid flow of cerebrospinal fluid from ventricles to cisterns via subarachnoid velae in the normal rat. Acta Neurochir Suppl 1997;70:285-7. [PubMed]
- Taketomo T, Saito A. Experimental studies on cerebrospinal fluid flow. Neurology 1965;15:578-86. [PubMed]
- Shapiro K, Kohn IJ, Takei F, et al. Progressive ventricular enlargement in cats in the absence of transmantle pressure gradients. J Neurosurg 1987;67:88-92. [PubMed]
- Stephensen H, Tisell M, Wikkelso C. There is no pressure gradient in communicating or non communicating hydrocephalus. Neurosurgery 2002;50:771-73.
- Brightman MW. The intracerebral movement of proteins injected into blood and cerebrospinal fluid of mice. In: Lajth A, Ford DH. eds. Progress in Brain Research, Brain Barrier System. Amsterdam: Elsevier, 1968:19-40.
- Cserr HF. Physiology of the choroid plexus. Physiol Rev 1971;51:273-311. [PubMed]
- Bulat M, Lupret V, Oreskovic D, et al. Transventricular and transpial absorption of cerebrospinal fluid into cerebral microvessels. Coll Antropol 2008;32:43-50. [PubMed]
- Weed LH. The dual source of CSF. J Med Res 1914;31:93-113. [PubMed]
- Dandy WE. Where is cerebrospinal fluid absorbed? JAMA 1929;92:2012-4.
- Brierly JF, Field EJ. The connections of the cerebrospinal fluid space with the lymphatic system. J Anat 1948;82:153-66.
- Bradbury M. Lymphatics and central nervous system. Trends Neurosci 1981;4:100-1.
- Johnston M, Zakharov A, Papaiconomou C, et al. Evidence of connections between cerebrospinal fluid and nasal lymphatic vessels in humans, non human primates and other mammalian species. Cerebrospinal Fluid Res 2004;1:2. [PubMed]
- Koh L, Zakharov A, Johnston M. Integration of the subarachnoid space and lymphatics; is it time to embrace a new concept of cerebrospinal fluid absorption? Cerebrospinal Fluid Res 2005;2:6-11. [PubMed]
- Maurizi CP. A cycle of cerebrospinal fluid. supporting evidence and theoretical considerations. Med Hypotheses 2000;54:417-22. [PubMed]
- Greitz D. Cerebrospinal fluid circulation and associated intracranial dynamics. A radiologic investigation using MR imaging and radionuclide cisternography. Acta Radiol Suppl 1993;386:1-23. [PubMed]
- Czosnyka M, Czosnyka Z, Momjian S, et al. Cerebrospinal fluid dynamics. Topical Review. Physiol Meas 2004;25:R51-76. [PubMed]
- Davson H. Formation and drainage of the cerebrospinal fluid. In: Shapiro K, Marmarou A, Portnoy H. eds. Hydrocephalus. New York: Raven Press, 1984:3-40.
- Jacobson EE, Fletcher DF, Morgan MK, et al. Fluid dynamics of cerebral aqueduct. Pediatr Neurosurg 1996;24:229-36. [PubMed]
- Penn RD, Lee MC, Linninger AA, et al. Pressure gradients in the brain in an experimental model of hydro-cephalus. J Neurosurg 2005;102:1069-75. [PubMed]
- Gato A, Moro JA, Alonso MI, et al. Chondroitin sulfate proteoglycan and embryonic brain enlargement in the chick. Anat Embryol 1993;188:101-6. [PubMed]
- Agre P. Aquaporin water channels. Biosci Rep 2004;24:127-63. [PubMed]
- Agre P, Nielsen S, Ottersen OP. Towards a molecular understanding of water homeostasis in the brain. Neuroscience 2004;129:849-50. [PubMed]
- Trinh-Trang-Tan MM, Cartron JP, Bankir L. Molecular basis for the dialysis disequilibrium syndrome. altered aquaporin and urea transporter expression in the brain. Nephrol Dial Transplant 2005;20:1984-8. [PubMed]
- Reinhold A, Zhang J, Gessner R, et al. High thrombopoietin concentrations in the cerebro- spinal fluid of neonates with sepsis and intraventricular hemorrhage may contribute to brain damage. J Interferon Cytokine Res 2007;27:137-45. [PubMed]
- Suzuki H, Muramatsu M, Tanaka K, et al. Cerebrospinal fluid ferritin in chronic hydrocephalus after aneurysmal subarachnoid hemorrhage. J Neurol 2006;253:1170-6. [PubMed]
- Chow LC, Soliman A, Zandian M, et al. Accumulation of transforming growth factor-beta2 and nitrated chondroitin sulfate proteoglycans in cerebrospinal fluid correlates with poor neurologic outcome in preterm hydrocephalus. Biol Neonate 2005;88:1-11. [PubMed]
- Flood C, Akinwunmi J, Lagord C, et al. TGF β1 in the CSF of patients with subarachnoid hemorrhage. titers derived from exogenous and endogenous sources. J Cereb Blood Flow Metab 2001;21:157-62. [PubMed]
- Whitelaw A, Christie S, Pople I. TGF β1: a possible signal molecule for posthemorrhagic hydrocephalus? Pediatr Res 1999;46:576-80. [PubMed]
- Heep A, Stoffel-Wagner B, Bartmann P, et al. Vascular endothelial growth factor and transforming growth factor-beta1 are highly expressed in the cerebrospinal fluid of premature infants with posthemorrhagic hydrocephalus. Pediatr Res 2004;56:768-74. [PubMed]
- Mashayekhi F, Salehi Z. Expression of nerve growth factor in cerebrospinal fluid of congenital hydrocephalic and normal children. Eur J Neurol 2005;12:632-7. [PubMed]
- Beems T, Simons KS, van Geel WJ, et al. Serum and CSF-concentrations of brain specific proteins in hydrocephalus. Acta Neurochir (Wien) 2003;145:37-43. [PubMed]
- Sendrowski K, Sobaniec W, Sobaniec-Lotowska ME, et al. S-100 protein as marker of the blood-brain barrier disruption in children with internal hydrocephalus and epilepsy--a preliminary study. Roczniki Akademii Medycznej W Bialymstoku 2004;49:236-8. [PubMed]
- Bloch J, Vernet O, Aube M, et al. Non-obstructive hydro- cephalus associated with intracranial schwannomas: hyper-proteinorrhachia as an etiopathological factor? Acta Neurochir (Wien) 2003;145:73-8. [PubMed]
- Dario A, Fachinetti P. Lumbar neurinoma associated with hydrocephalus. Case report. J Neurosurg Sci 1993;37:179-82. [PubMed]
- Ohta K, Gotoh F, Amando T, et al. Normal pressure hydrocephalus associated with cauda equina neurinoma. Ann Neurol 1990;27:441-3. [PubMed]
- Erşahin Y, Mutler S, Yurtseven T. Hydrocephalus in Guillain-Barre’ syndrome. Clin Neurol Neurosurg 1995;97:253-5. [PubMed]
- Ropper AH, Marmarou A. Mechanism of pseudotumor in Guillain-Barre’ syndrome. Arch Neurol 1984;41:259-61. [PubMed]
- Tarnaris A, Watkins LD, Kitchen ND. Biomarkers in chronic adult hydrocephalus. Cerebrospinal Fluid Res 2006;3:11. [PubMed]
- Nooijen PT, Schoonderwaldt HC, Wevers RA, et al. Neuronspecific enolase, S-100 protein, myelin basic protein and lactate in CSF in dementia. Dement Geriatr Cogn Disord 1997;8:169-73. [PubMed]
- Nussinovitch M, Volovitz B, Finkelstein Y, et al. Lactic dehydrogenase isoenzymes in cerebrospinal fluid associated with hydrocephalus. Acta Paediatr 2001;90:972-4. [PubMed]
- Cerda M, Manterola A, Ponce S, et al. Electrolyte levels in the CSF of children with non-tumoral hydrocephalus. Childs Nerv Syst 1985;1:306-11. [PubMed]
- Del Bigio MR. Hydrocephalus-induced changes in the composition of cerebrospinal fluid. Neurosurgery 1989;25:416-23. [PubMed]
- Whitelaw A, Pople I, Cherian S, et al. Phase I trial of prevention of hydrocephalus after intraventricular hemorrhage in newborn infants by drainage, irrigation and fibrinolytic therapy. Pediatrics 2003;111:759-65. [PubMed]
- Peretta P, Regazzi P, Carlino CF, et al. The role of Ommaya reservoir and endoscopic third ventriculostomy in the management of post hemorrhagic hydrocephalus in prematurity. Childs Nerv Syst 2007;23:765-71. [PubMed]
- Brinker T, Seifert V, Dietz H. Subacute hydrocephalus after experimental subarachnoid hemorrhage: its prevention by intrathecal fibrinolysis with recombinant tissue plasminogen activator. Neurosurgery 1992;31:306-11. [PubMed]
- Wald A, Hochwald GM, Malhan C. The effects of ventricular fluid osmolality on bulk flow of nascent fluid into the cerebral ventricles of cats. Exp Brain Res 1976;25:157-67. [PubMed]
- DiMattio J, Hochwald GM, Malhan C, et al. Effects of changes in serum osmolality on bulk flow of fluid into cerebral ventricles and on brain water content. Pflugers Arch 1975;359:253-64. [PubMed]
- Johanson CE, Szmydynger-Chobodska J, Chobodski A, et al. Altered formation and bulk absorption of cerebrospinal fluid in FGF-2 induced hydrocephalus. Am J Physiol 1999;277:R263-71. [PubMed]
- Gao F, Liu F, Chen Z, et al. Hydrocephalus after intraventricular hemorrhage. the role of thrombin. J Cereb Blood Flow Metab 2014;34:489-94. [PubMed]
- Krishnamurthy S, Li J, Schultz L, et al. Intraventricular infusion of hyperosmolar dextran induces hydrocephalus; a novel animal model of hydrocephalus. Cerebrospinal Fluid Research 2009;6:16. [PubMed]
- Krishnamurthy S, Li J, Schultz L, et al. Increased CSF osmolarity reversibly induces hydrocephalus in the normal rat brain. Fluids Barriers CNS 2012;9:13. [PubMed]
- Lowery LA, Sive H. Initial formation of zebrafish brain ventricles occurs independently of circulation and requires the nagieoko and snakehead/atp1a1a.1 gene products. Development 2005;132:2057-67. [PubMed]
- Parvas M, Bueno D. The Embryonic Blood-CSF Barrier has Molecular Elements to Control E-CSF Osmolarity During early CNS Development. J Neurosci Res 2010;88:1205-12. [PubMed]
- Klarica M, Oreskovic D, Kalousek M, et al. Intracranial pressure response to application of hyperosmolal sucrose into cerebrospinal fluid by the microvolume exchange method in dogs. Neurol Croat 1994;43:147-54.
- Alonso MI, Gato A, Moro JA, et al. Disruption of proteoglycans in neural tube fluid by β-D xyloside alters brain enlargement in chick embryos. Anat Rec 1998;252:499-508. [PubMed]
- Wagner HJ, Pilgrim CH, Brandl J. Penetration and removal of horseradish peroxidase injected into the cerebral spinal fluid; role of cerebral perivascular spaces, endothelium and microglia. Acta Neuropathol 1974;27:299-315. [PubMed]
- Rennels ML, Gregory TF, Blaumanis OR, et al. Evidence for a “paravascular” fluid circulation in the mammalian central nervous system, provided by the rapid distribution of tracer protein throughout the brain from the subarachnoid space. Brain Res 1985;326:47-63. [PubMed]
- Blaumanis OR, Rennels ML, Grady PA. Focal cerebral edema impedes convective fluid/tracer movement through paravascular pathways in cat brain. Adv Neurol 1990;52:385-9. [PubMed]
- Zhang ET, Richards HK, Kida S, et al. Directional and compartmentalized drainage of interstitial fluid and cerebrospinal fluid from the rat brain. Acta Neuropathol 1992;83:233-9. [PubMed]
- Iliff JJ, Wang M, Liao Y, et al. A paravascular pathway facilitates CSF flow through the brain parenchyma and the clearance of interstitial solutes, including amyloid β. Sci Transl Med 2012;4:147ra111.
- Iliff JJ, Lee H, Yu M, et al. Brain wide pathway for waste clearance captured by contrast enhanced MRI. J Clin Invest 2013;123:1299-309. [PubMed]
- Weller RO, Kida S, Zhang ET. Pathways of fluid drainage from the brain-morphological aspects and immunological significance in rat and man. Brain Pathol 1992;2:277-84. [PubMed]
- Williams H. The venous hypothesis of hydrocephalus. Med Hypotheses 2008;70:743-47. [PubMed]
- Williams H. A unifying hypothesis for hydrocephalus, Chiari malformation, syringomyelia, anencephaly and spina bifida. Cerebrospinal Fluid Research 2008;5:7. [PubMed]
- Caplan LR. Cardiac encephalopathy and congestive heart failure: a hypothesis about the relationship. Neurology 2006;66:99-101. [PubMed]
- Collmann H, Sörensen N, Krauss J. Hydrocephalus in craniosynostosis: a review. Childs Nerv Syst 2005;21:902-12. [PubMed]
- Ebinu JO, Matouk CC, Wallace MC, et al. Hydrocephalus secondary to hydrodynamic disequilibrium in an adult patient with a choroidal type arteriovenous malformation. Interv Neuroradiol 2011;17:212-6. [PubMed]
- Geibprasert S, Pereira V, Krings T, et al. Hydrocephalus in unruptured brain arteriovenous malformations: Pathomechanical considerations, therapeutic implications, and clinical course. J Neurosurg 2009;110:500-7. [PubMed]
- Lobato RD, Lamas E, Cordobes F, et al. Chronic adult hydrocephalus due to uncommon causes. Acta Neurochir (Wien) 1980;55:85-97. [PubMed]