Energy metabolism in neurodevelopment and medulloblastoma
Introduction
Metabolically-targeted therapies hold the promise of offering newly effective, less toxic treatment for medulloblastoma, the most common malignant brain tumor of childhood. Current therapy relies on the sensitivity of medulloblastoma to DNA damage that was discovered more than 50 years ago. Craniospinal radiation therapy, first implemented in the 1950s, changed medulloblastoma from a uniformly fatal disease into a treatable cancer with 60% long-term survival (1). Since then, decades of clinical trials have produced incremental improvement in outcomes by adding chemotherapy, with 80% of standard risk patients and 70% of high risk patients surviving more than 5 years (2-6). The success of radiation and chemotherapy however, comes at significant costs, as survivors must live with untoward effects of treatment, including dementia, early strokes, growth impairment and hearing loss (7-14). Survivors also remain at risk of recurrence, which is presently incurable, and 20-30% of medulloblastoma patients eventually die of disease (2,15,16). Finding new tumor-specific vulnerabilities to complement sensitivity to DNA damage may allow new approaches to therapy. Recent investigations suggest that the metabolic program of medulloblastoma may be a previously untested vulnerability.
Here, we review specific features of medulloblastoma metabolism that may be targeted therapeutically. Some metabolic processes typical of medulloblastoma, including increased lipogenesis and aerobic glycolysis, derive from neural development. Other metabolic processes, including global inhibition of protein translation, may not have a clear developmental correlate but rather may be common features of cancer. We propose that these diverse metabolic processes are interrelated, as shown in Figures 1,2, and may be targeted in concert to maximize the potential anti-tumor effect.
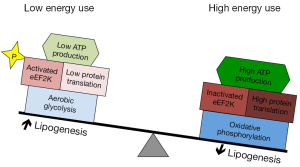
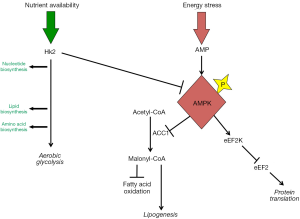
Neural progenitors and medulloblastoma cells share distinct metabolic needs
Medulloblastoma is a molecularly heterogeneous set of tumors that arise from the cerebellum, share common histologic patterns, but arise from different sets of neural progenitors. Two molecular subgroups of medulloblastoma have been related to hyperactivation developmental signaling pathways, specifically Sonic Hedgehog (Shh) and WNT. Lineage tracing in transgenic mice demonstrates that activation of these pathways transforms distinct but related sets of neural progenitors. Shh hyperactivation drives medulloblastoma formation specifically in cerebellar granule neuron progenitors (CGNPs), a population that derives from the upper rhombic lip (17,18). WNT pathway activation, combined with p53 deletion, drives medulloblastoma formation from brain stem progenitors that derive from the lower rhombic lip (19). The mitotic effect of Shh on CGNPs and the oncogenic effect of Shh-pathway hyperstimulation provide an ideal experimental platform to study metabolic patterns as neural progenitors proliferate and give rise to progenitor—derived tumors.
Both neural progenitors and tumor cells face metabolic challenges that distinguish them from other cells in the brain. All cells consume energy as they maintain homeostasis, and all cells in the brain produce energy from glucose, ketones and amino acids. Neurons, which are post-mitotic cells, are the largest energy consumers in the brain, expending energy to maintain electrical activity and intercellular communication (20,21). The efficiency of ATP generation may therefore be of paramount importance. The need for efficient ATP generation in proliferating cells in the brain, however, may be balanced by additional metabolic needs.
Unlike post-mitotic neurons, proliferative cells must not only extract energy from substrates, but also metabolize these substrates into the nucleic acids, proteins and lipids for their progeny (22). In the first 2 post-natal weeks in mice, and in the first year of life in humans, the cerebellum is the site of rapid proliferation, as CGNPs divide in response to Shh signaling. CGNP proliferation generates the largest population of neurons in the brain. For the highly proliferative CGNPs, the generation of intermediates for the synthesis of lipids, nucleic acids and proteins may compete for priority with the downstream generation of ATP. Cells of high-grade tumors, including medulloblastoma and glioblastoma, must similarly balance energy metabolism with the need to synthesize the macromolecules essential for tumor growth. Lower grade tumors, in contrast, may not require constant accumulation of biomass, and can therefore prioritize ATP production. The different metabolic needs of proliferative and non-proliferative cell populations are matched by profound differences in their mechanisms of nutrient metabolism.
Neural progenitor cells configure metabolism to support lipogenesis
In concert with the induction of proliferation, Shh induces lipogenesis in CGNPs through a mechanism that is preserved in medulloblastoma (23-25). At the level of transcriptional regulation, Shh pathway activation up-regulates proteins required for lipid synthesis, including fatty acid synthase (FASN) and acetyl-CoA carboxylase 1 (ACC1), and down-regulates fatty acid catabolism enzymes, including acyl-CoA oxidase 1 (ACOX1) and medium chain acyl-CoA dehydrogenase (MCAD). These transcriptional changes are mirrored by changes in the metabolic activity of CGNPs, demonstrated in vitro where Shh decreases CGNP fatty acid oxidation (24). The transcriptional regulation of lipid metabolism enzymes in CGNPs is coordinated by Shh-dependent activation of E2F1 via Rb modulation (24). Through this system, Shh induces both increased cell cycling and increased lipogenesis, thus ensuring that macromolecule biosynthesis is coupled with proliferation. Likewise, Shh further induces changes in glucose metabolism to support biosynthesis.
Aerobic glycolysis allows neural progenitors to balance energy and biosynthesis requirements
Glucose is the primary energy source in the brain. Through oxidative phosphorylation, glucose may be metabolized to H2O and CO2 to generate 38 ATP per molecule. Alternatively, glucose may be metabolized to pyruvate through glycolysis, ultimately generating lactate and 2 ATP. Aerobic glycolysis is the metabolism of glucose to lactate despite the presence of sufficient oxygen needed for oxidative phosphorylation. Cells with large ATP requirements are likely to be disadvantaged by aerobic glycolysis because glycolysis generates less ATP per molecule of glucose than oxidative phosphorylation. Proliferating cells, however, may use aerobic glycolysis to satisfy the competing needs for both energy generation and the accumulation of biomass (Figure 1). Unlike oxidative phosphorylation, which converts glucose to H2O and CO2, aerobic glycolysis also generates metabolic intermediates that can be used for lipid and nucleic acid biosynthesis (26). Consistent with their different energy requirements, neurons and neural progenitors use specific sets of glucose metabolizing enzymes that promote either oxidative phosphorylation or aerobic glycolysis.
Differentiated neurons and neural progenitors metabolize glucose using distinct sets of glycolytic enzymes. Hexokinases catalyze glucose phosphorylation, the first modification of glucose upon entry into the cell. There are four mammalian genes coding for hexokinases (Hk1-4). Hk1 is expressed by neurons and glia throughout the brain but excluded from regions of neural progenitors (27). In contrast, Shh signaling induces Hk2 expression in CGNPs (27). Further downstream in the glycolytic pathway, Shh induces splicing of the enzyme pyruvate kinase into the M2 isoform (PkM2) (23,28).
In diverse cell types, Hk2 and PkM2 are associated with aerobic glycolysis and metabolomic analysis demonstrates that Shh induces aerobic glycolysis in CGNPs (23,27). Cerebellar glucose uptake and lactate generation are specifically increased during the period of postnatal cerebellar neurogenesis, and CGNPs cultured in the presence of Shh increase glucose uptake and lactate generation, without increasing oxygen consumption (27). Genetic deletion of Hk2 in CGNPs blocked Shh-induced aerobic glycolysis, demonstrating the central role of Hk2 in configuring CGNPmetabolism in response to Shh signaling.
The expression of PkM2 in response to Shh suggests that this isozyme also plays an important role in CGNP growth and metabolism. PkM2 has been widely held to be an oncogene because it is ubiquitously expressed in cancer. A seminal study comparing xenografts engineered to express either PkM1 or PkM2 showed the M2 isoform conferred a growth advantage (29). Since then, a variety of oncogenic mechanisms have been attributed to PkM2, including metabolic reprogramming, histone phosphorylation and direct regulation of gene expression (30-33). More recently, PkM2 has been proposed to promote proliferation by acting as a regulated, inefficient converter of phosphoenolpyruvate (PEP) to pyruvate (34). In response to external and internal signals, such as changes in nutrient availability, PkM2 activity can be modulated to fulfill metabolic demands. Down-regulating the catalytic activity of PkM2 slows the flow of PEP through glycolysis, increases the supply of intermediates upstream of PEP, and allows for alternative uses of PEP, including the activating phosphorylation of the enzyme phosphoglycerate mutase 1 (Pgam1) (35). Pgam1 in turn may promote the shunting of intermediates into anabolic pathways through its effects on the balance of 2- and 3-phosphoglycerate, which feedback on serine synthesis and the pentose phosphate pathway (36). Through these mechanisms, PkM2 may increase the efficiency with which aerobic glycolysis fulfills the biosynthetic needs of CGNPs.
Increased lipogenesis and aerobic glycolysis in medulloblastoma
Malignant brain tumor cells, like neural progenitors, are highly proliferative and must meet the competing needs to generate energy through nutrient catabolism and to convert nutrients into macromolecules necessary for growth. The transcriptional regulators and enzymes that mediate increased lipogenesis and aerobic glycolysis in Shh-stimulated CGNPs also control the metabolism of tumor cells in mouse models of Shh-driven medulloblastoma. The ND2:SmoA1 and SmoM2 mouse lines, express different, constitutively active alleles of the Shh effector Smoothened and develop spontaneous medulloblastomas. These tumors demonstrate up-regulation of FASN, E2F1 activation and deposition of lipid droplets, indicating a high rate of lipid synthesis (24), and up-regulation of Hk2 and PkM2, consistent with increased aerobic glycolysis (23,27). The glycolytic metabolism of medulloblastoma is further demonstrated by 18FDG PET studies that show intense glucose avidity of medulloblastomas in both mice and humans, even when compared to the typically high glucose uptake of the brain (27,37).
Aerobic glycolysis and lipogenesis as common features across brain tumor types
While the aerobic glycolysis and lipogenesis have been most extensively studied in Shh-driven medulloblastoma, these metabolic patterns may be common across medulloblastoma subgroups. Whether FDG avidity in the human disease varies with subtype is not known. WNT pathway activation has been shown to increase glycolysis at the expense of oxidative phosphorylation in both normal and transformed cells outside the brain (38,39). The MYC family of transcription factors is known to up-regulate glycolysis in diverse cancers (40-42) and several lines of evidence suggest that MYC activation may be a key point of convergence that shapes medulloblastoma metabolism across subgroups. MycN operates downstream of Shh to drive aerobic glycolysis in CGNPs and Shh-driven medulloblastoma in SmoM2 mice. WNT signaling may also promote glycolysis in part through MYC (43); whether this relationship operates in WNT-subgroup medulloblastomas remains to be tested. Group 3 medulloblastomas are associated with MYC-family amplifications and MycN overexpression in mice produces medulloblastomas that resemble group 3 tumors in gene expression pattern (44,45). While glucose metabolism has not yet been examined in group 3 medulloblastoma or in MycN-driven medulloblastoma in mice, it is likely that in these tumors, as in other cancers, MYC reconfigures metabolism to favor glycolysis. Importantly, aerobic glycolysis plays a key role in malignant brain tumors with diverse pathologies, as glioblastomas like medulloblastomas express Hk2 (46) and demonstrate high glucose uptake in 18FDG PET studies (47,48). In the activation of lipogenesis and aerobic glycolysis, diverse malignant brain tumors recapitulate the metabolic adaptions of neural progenitors.
Disruption of lipogenesis or glycolysis restricts brain tumor growth
Experimental evidence shows that E2F1-mediated lipogenesis and Hk2-dependent glycolysis play important roles in supporting medulloblastoma progression. In SmoA1 mice, treatment with either the CDK inhibitor olomoucine or the FASN inhibitor C75 significantly inhibited tumor growth and extended animal survival (24). Similarly, genetic deletion of Hk2 in medulloblastoma-prone GFAP-cre:SmoM2 mice significantly inhibited tumor growth. GFAP-cre:SmoM2 mice are a highly penetrant, rapidly progressive, primary medulloblastoma model ideally suited for genetic studies. These mice express a constitutively active allele of the Shh receptor component smoothened throughout the brain and die from medulloblastoma with 100% frequency by postnatal day 20 (18). Genetic deletion of Hk2 in GFAP-cre:SmoM2 mice markedly reduced the aggressiveness of the resulting medulloblastomas, causing tumors to grow as indolent lesions and allowing long-term survival of tumor-bearing mice (27). Importantly, Hk2 deletion was associated in tumors with activation of the intracellular energy regulator protein adenosine monophosphate-activated protein kinase (AMPK), which inhibits ACC1; this feedback through AMPK may operate alongside the generation of metabolites to link aerobic glycolysis to lipogenesis. In glioblastoma xenografts, Hk2 depletion similarly limited tumor growth and increased treatment sensitivity, while Hk2 overexpression enhanced tumor progression (46). Taken together, these results suggest that inhibiting the metabolic adaptations common to neural progenitors and brain tumors may be a novel approach to brain tumor therapy. The integration of glycolysis and lipogenesis in progenitors suggests that targeting both processes in combination may reinforce the anti-tumor effect.
Restriction of energy expenditure in medulloblastoma enables tumor metabolism
Mechanisms that limit energy consumption enable tumors to maximize lipogenesis and to benefit optimally from aerobic glycolysis. While metabolizing glucose through glycolysis supports the channeling of nutrients into biosynthesis, the inefficient production of ATP presents a risk if nutrient availability is compromised. Minimizing tumor energy consumption may mitigate this risk. The net conversion of glucose to lipid is also a liability unless energy needs can be lessened. A mechanism for limiting tumor energy expenditure by inhibiting mRNA translation has recently been described and found to be active in medulloblastoma (49). This mechanism may be essential in allowing tumor cells to fully engage in the lipogenic and glycolytic mechanisms of neural progenitors without the liability of energy scarcity (Figure 1).
The eukaryotic elongation factor eukaryotic elongation factor 2 (eEF2) plays an essential role in translation by promoting GTP-dependent translocation of the growing peptide chain along the ribosome (50). The activity of eEF2 is down-regulated through phosphorylation by eukaryotic elongation factor 2 kinase (eEF2K) (51). In turn, eEF2K is activated by the energy sensor AMPK (52,53). Expression of eEF2K correlates negatively with patient survival in both medulloblastoma and glioblastoma, suggesting that reducing eEF2 activity promotes tumor growth (49). Consistent with this interpretation, disruption of eEF2K expression in xenografted cell lines sensitized tumors to nutrient deprivation, causing tumors in mice fed calorie-restricted diets to grow more slowly and with increased tumor cell death (49).
Combinatorial targeting of tumor metabolism
Reducing energy expenditure through eEF2K-mediated translation inhibition enables tumor cells to increase aerobic glycolysis, which in turn supports lipogenesis. Simultaneously targeting each of these processes may maximize stress to the tumor cell. While individually disrupting Hk2 or FASN slowed tumor growth, neither intervention matched the strong response induced by radiation. Specific inhibitors of Hk2, FASN and eEF2K may produce synergistic effects, however, and should be tested experimentally in combination in order to maximize potential effect. Similarly, a ketogenic diet may effectively target both lipogenesis and glycolysis by reducing nutrient availability for these pathways. A high-fat, low carbohydrate diet shifts the primary energy substrate for energy metabolism away from glucose to ketone bodies generated from fatty acid catabolism. Normal brain metabolism inherently relies on ketone bodies as an alternative energy source, thus a shift away from glucose metabolism may induce energy stress on the glucose-dependent tumor while minimally affecting energy balance in the normal brain (54). While the implementation of a ketogenic diet as a therapy for medulloblastoma has not been described, case reports describe modest benefits for patients with glioblastoma (55-58). A more robust effect may result from combination with eEF2K inhibition.
Intriguingly, the intracellular energy sensor protein AMPK represents a point of convergence between these different processes (Figure 2). AMPK exerts a homeostatic effect by integrating lipogenesis, energy production and protein translation. Targeting AMPK may be problematic however, as activation and inhibition of AMPK have the potential for mixed effects on growth. AMPK activation inhibits lipogenesis through inhibitory phosphorylation of ACC1 (59-61), which may restrict tumor growth. Consistent with a tumor suppressive effect, Hk2 deletion produced AMPK activation in GFAP-cre:SmoM2 medulloblastomas while slowing tumor growth (27). AMPK activation, however, also phosphorylates eEF2K, which may promote growth by limiting energy expenditure (51,62). In order to disrupt the homeostatic effect of AMPK-mediated integration, it may be most effective to directly target simultaneously lipogenesis, eEF2K and aerobic glycolysis. A ketogenic diet combined with specific inhibitors of Hk2 and/or eEF2K may achieve this goal and optimally target tumor metabolism.
Acknowledgements
Funding: Timothy R. Gershon is supported by grants from the National Institutes of Health (NIH; 1K08NS077978-01), the St. Baldrick’s Foundation, and the American Institute for Cancer Research.
Footnote
Conflicts of Interest: The authors have no conflicts of interest to declare.
References
- Paterson E, Farr RF. Cerebellar medulloblastoma: treatment by irradiation of the whole central nervous system. Acta radiol 1953;39:323-36. [PubMed]
- Packer RJ, Gajjar A, Vezina G, et al. Phase III study of craniospinal radiation therapy followed by adjuvant chemotherapy for newly diagnosed average-risk medulloblastoma. J Clin Oncol 2006;24:4202-8. [PubMed]
- Packer RJ, Goldwein J, Nicholson HS, et al. Treatment of children with medulloblastomas with reduced-dose craniospinal radiation therapy and adjuvant chemotherapy: A Children's Cancer Group Study. J Clin Oncol 1999;17:2127-36. [PubMed]
- Evans AE, Jenkin RD, Sposto R, et al. The treatment of medulloblastoma. Results of a prospective randomized trial of radiation therapy with and without CCNU, vincristine, and prednisone. J Neurosurg 1990;72:572-82. [PubMed]
- Thomas PR, Deutsch M, Kepner JL, et al. Low-stage medulloblastoma: final analysis of trial comparing standard-dose with reduced-dose neuraxis irradiation. J Clin Oncol 2000;18:3004-11. [PubMed]
- Packer RJ, Sutton LN, Elterman R, et al. Outcome for children with medulloblastoma treated with radiation and cisplatin, CCNU, and vincristine chemotherapy. J Neurosurg 1994;81:690-8. [PubMed]
- Schreiber JE, Gurney JG, Palmer SL, et al. Examination of risk factors for intellectual and academic outcomes following treatment for pediatric medulloblastoma. Neuro Oncol 2014;16:1129-36. [PubMed]
- Ris MD, Walsh K, Wallace D, et al. Intellectual and academic outcome following two chemotherapy regimens and radiotherapy for average-risk medulloblastoma: COG A9961. Pediatr Blood Cancer 2013;60:1350-7. [PubMed]
- Lafay-Cousin L, Purdy E, Huang A, et al. Early cisplatin induced ototoxicity profile may predict the need for hearing support in children with medulloblastoma. Pediatr Blood Cancer 2013;60:287-92. [PubMed]
- Vieira WA, Weltman E, Chen MJ, et al. Ototoxicity evaluation in medulloblastoma patients treated with involved field boost using intensity-modulated radiation therapy (IMRT): a retrospective review. Radiat Oncol 2014;9:158. [PubMed]
- Grenier Y, Tomita T, Marymont MH, et al. Late postirradiation occlusive vasculopathy in childhood medulloblastoma. Report of two cases. J Neurosurg 1998;89:460-4. [PubMed]
- Bansal LR, Belair J, Cummings D, et al. Late-Onset Radiation-Induced Vasculopathy and Stroke in a Child With Medulloblastoma. J Child Neurol 2014. [Epub ahead of print]. [PubMed]
- Salih IS, Higgins NJ, Warburton EA, et al. Lacunar stroke attributable to radiation-induced intracranial arteriopathy. Eur J Neurol 2007;14:937-9. [PubMed]
- Maher CO, Raffel C. Early vasculopathy following radiation in a child with medulloblastoma. Pediatr Neurosurg 2000;32:255-8. [PubMed]
- Tarbell NJ, Friedman H, Polkinghorn WR, et al. High-risk medulloblastoma: a pediatric oncology group randomized trial of chemotherapy before or after radiation therapy (POG 9031). J Clin Oncol 2013;31:2936-41. [PubMed]
- Jakacki RI, Burger PC, Zhou T, et al. Outcome of children with metastatic medulloblastoma treated with carboplatin during craniospinal radiotherapy: a Children's Oncology Group Phase I/II study. J Clin Oncol 2012;30:2648-53. [PubMed]
- Yang ZJ, Ellis T, Markant SL, et al. Medulloblastoma can be initiated by deletion of Patched in lineage-restricted progenitors or stem cells. Cancer Cell 2008;14:135-45. [PubMed]
- Schüller U, Heine VM, Mao J, et al. Acquisition of granule neuron precursor identity is a critical determinant of progenitor cell competence to form Shh-induced medulloblastoma. Cancer Cell 2008;14:123-34. [PubMed]
- Gibson P, Tong Y, Robinson G, et al. Subtypes of medulloblastoma have distinct developmental origins. Nature 2010;468:1095-9. [PubMed]
- Howarth C, Gleeson P, Attwell D. Updated energy budgets for neural computation in the neocortex and cerebellum. J Cereb Blood Flow Metab 2012;32:1222-32. [PubMed]
- Mergenthaler P, Lindauer U, Dienel GA, et al. Sugar for the brain: the role of glucose in physiological and pathological brain function. Trends Neurosci 2013;36:587-97. [PubMed]
- Vander Heiden MG, Cantley LC, Thompson CB. Understanding the Warburg effect: the metabolic requirements of cell proliferation. Science 2009;324:1029-33. [PubMed]
- Bhatia B, Potts CR, Guldal C, et al. Hedgehog-mediated regulation of PPARγ controls metabolic patterns in neural precursors and shh-driven medulloblastoma. Acta Neuropathol 2012;123:587-600. [PubMed]
- Bhatia B, Hsieh M, Kenney AM, et al. Mitogenic Sonic hedgehog signaling drives E2F1-dependent lipogenesis in progenitor cells and medulloblastoma. Oncogene 2011;30:410-22. [PubMed]
- Tech K, Deshmukh M, Gershon TR. Adaptations of energy metabolism during cerebellar neurogenesis are co-opted in medulloblastoma. Cancer Lett 2015;356:268-72. [PubMed]
- Lunt SY, Vander Heiden MG. Aerobic glycolysis: meeting the metabolic requirements of cell proliferation. Annu Rev Cell Dev Biol 2011;27:441-64. [PubMed]
- Gershon TR, Crowther AJ, Tikunov A, et al. Hexokinase-2-mediated aerobic glycolysis is integral to cerebellar neurogenesis and pathogenesis of medulloblastoma. Cancer Metab 2013;1:2. [PubMed]
- Di Magno L, Manzi D, D'Amico D, et al. Druggable glycolytic requirement for Hedgehog-dependent neuronal and medulloblastoma growth. Cell Cycle 2014;13:3404-13. [PubMed]
- Christofk HR, Vander Heiden MG, Harris MH, et al. The M2 splice isoform of pyruvate kinase is important for cancer metabolism and tumour growth. Nature 2008;452:230-3. [PubMed]
- Lu Z. PKM2 functions as a histone kinase. Cell Cycle 2012;11:4101-2. [PubMed]
- Yang W, Xia Y, Ji H, et al. Nuclear PKM2 regulates β-catenin transactivation upon EGFR activation. Nature 2011;480:118-22. [PubMed]
- Yang W, Zheng Y, Xia Y, et al. ERK1/2-dependent phosphorylation and nuclear translocation of PKM2 promotes the Warburg effect. Nat Cell Biol 2012;14:1295-304. [PubMed]
- Yang W, Lu Z. Regulation and function of pyruvate kinase M2 in cancer. Cancer Lett 2013;339:153-8. [PubMed]
- Vander Heiden MG, Lunt SY, Dayton TL, et al. Metabolic pathway alterations that support cell proliferation. Cold Spring Harb Symp Quant Biol 2011;76:325-34. [PubMed]
- Vander Heiden MG, Locasale JW, Swanson KD, et al. Evidence for an alternative glycolytic pathway in rapidly proliferating cells. Science 2010;329:1492-9. [PubMed]
- Hitosugi T, Zhou L, Elf S, et al. Phosphoglycerate mutase 1 coordinates glycolysis and biosynthesis to promote tumor growth. Cancer Cell 2012;22:585-600. [PubMed]
- Gururangan S, Hwang E, Herndon JE 2nd, et al. [18F]fluorodeoxyglucose-positron emission tomography in patients with medulloblastoma. Neurosurgery 2004;55:1280-8; discussion 1288-9. [PubMed]
- Esen E, Chen J, Karner CM, et al. WNT-LRP5 signaling induces Warburg effect through mTORC2 activation during osteoblast differentiation. Cell Metab 2013;17:745-55. [PubMed]
- Pate KT, Stringari C, Sprowl-Tanio S, et al. Wnt signaling directs a metabolic program of glycolysis and angiogenesis in colon cancer. EMBO J 2014;33:1454-73. [PubMed]
- Wang R, Dillon CP, Shi LZ, et al. The transcription factor Myc controls metabolic reprogramming upon T lymphocyte activation. Immunity 2011;35:871-82. [PubMed]
- Dang CV, Le A, Gao P. MYC-induced cancer cell energy metabolism and therapeutic opportunities. Clin Cancer Res 2009;15:6479-83. [PubMed]
- Dang CV. MYC on the path to cancer. Cell 2012;149:22-35. [PubMed]
- Thompson CB. Wnt meets Warburg: another piece in the puzzle? EMBO J 2014;33:1420-2. [PubMed]
- Kool M, Korshunov A, Remke M, et al. Molecular subgroups of medulloblastoma: an international meta-analysis of transcriptome, genetic aberrations, and clinical data of WNT, SHH, Group 3, and Group 4 medulloblastomas. Acta Neuropathol 2012;123:473-84. [PubMed]
- Pöschl J, Stark S, Neumann P, et al. Genomic and transcriptomic analyses match medulloblastoma mouse models to their human counterparts. Acta Neuropathol 2014;128:123-36. [PubMed]
- Wolf A, Agnihotri S, Micallef J, et al. Hexokinase 2 is a key mediator of aerobic glycolysis and promotes tumor growth in human glioblastoma multiforme. J Exp Med 2011;208:313-26. [PubMed]
- Goda JS, Dutta D, Raut N, et al. Can multiparametric MRI and FDG-PET predict outcome in diffuse brainstem glioma? A report from a prospective phase-II study. Pediatr Neurosurg 2013;49:274-81. [PubMed]
- Padma MV, Said S, Jacobs M, et al. Prediction of pathology and survival by FDG PET in gliomas. J Neurooncol 2003;64:227-37. [PubMed]
- Leprivier G, Remke M, Rotblat B, et al. The eEF2 kinase confers resistance to nutrient deprivation by blocking translation elongation. Cell 2013;153:1064-79. [PubMed]
- Kaul G, Pattan G, Rafeequi T. Eukaryotic elongation factor-2 (eEF2): its regulation and peptide chain elongation. Cell Biochem Funct 2011;29:227-34. [PubMed]
- Ryazanov AG, Shestakova EA, Natapov PG. Phosphorylation of elongation factor 2 by EF-2 kinase affects rate of translation. Nature 1988;334:170-3. [PubMed]
- Horman S, Browne G, Krause U, et al. Activation of AMP-activated protein kinase leads to the phosphorylation of elongation factor 2 and an inhibition of protein synthesis. Curr Biol 2002;12:1419-23. [PubMed]
- Browne GJ, Finn SG, Proud CG. Stimulation of the AMP-activated protein kinase leads to activation of eukaryotic elongation factor 2 kinase and to its phosphorylation at a novel site, serine 398. J Biol Chem 2004;279:12220-31. [PubMed]
- Veech RL. The therapeutic implications of ketone bodies: the effects of ketone bodies in pathological conditions: ketosis, ketogenic diet, redox states, insulin resistance, and mitochondrial metabolism. Prostaglandins Leukot Essent Fatty Acids 2004;70:309-19. [PubMed]
- Stafford P, Abdelwahab MG. The ketogenic diet reverses gene expression patterns and reduces reactive oxygen species levels when used as an adjuvant therapy for glioma. Nutr Metab (Lond) 2010;7:74. [PubMed]
- Nebeling LC, Miraldi F, Shurin SB, et al. Effects of a ketogenic diet on tumor metabolism and nutritional status in pediatric oncology patients: two case reports. J Am Coll Nutr 1995;14:202-8. [PubMed]
- Zhou W, Mukherjee P, Kiebish MA, et al. The calorically restricted ketogenic diet, an effective alternative therapy for malignant brain cancer. Nutr Metab (Lond) 2007;4:5. [PubMed]
- Seyfried TN, Kiebish M, Mukherjee P, et al. Targeting energy metabolism in brain cancer with calorically restricted ketogenic diets. Epilepsia 2008;49 Suppl 8:114-6. [PubMed]
- Hardie DG, Pan DA. Regulation of fatty acid synthesis and oxidation by the AMP-activated protein kinase. Biochem Soc Trans 2002;30:1064-70. [PubMed]
- Carling D, Zammit VA, Hardie DG. A common bicyclic protein kinase cascade inactivates the regulatory enzymes of fatty acid and cholesterol biosynthesis. FEBS Lett 1987;223:217-22. [PubMed]
- Kemp BE, Mitchelhill KI, Stapleton D, et al. Dealing with energy demand: the AMP-activated protein kinase. Trends Biochem Sci 1999;24:22-5. [PubMed]
- Carlberg U, Nilsson A, Nygård O. Functional properties of phosphorylated elongation factor 2. Eur J Biochem 1990;191:639-45. [PubMed]