Potential therapeutics in pediatric acute respiratory distress syndrome: what does the immune system have to offer? A narrative review
Acute respiratory distress syndrome (ARDS) is characterized by acute onset of diffuse bilateral pulmonary edema and severe hypoxemia not fully explained by cardiac dysfunction (1-3). Since its initial description in 1967 (3), ARDS has been considered an immune-mediated phenomenon. ARDS is triggered most commonly by pulmonary and non-pulmonary infections, with a minority of cases caused by aspiration, trauma, pancreatitis, and drug reactions (4). Linking these diverse infectious and non-infectious etiologies to a final common manifestation of ARDS has been the subject of intensive investigation over the past 50 years.
ARDS affects 45,000 children in the United States annually (5), representing 10% of invasively ventilated children (6), with an associated 20% mortality rate (7-10). Until recently, ARDS had been defined exclusively by adult investigators predominantly for adult practitioners. To inform study design in children with ARDS, the Pediatric Acute Lung Injury Consensus Conference (PALICC) developed a specific definition for pediatric ARDS (PARDS) in 2015 (11). Notable differences in the PALICC definition include use of oxygenation index (rather than PaO2/FIO2) for severity stratification, explicit use of alternative stratification using SpO2, and inclusion of unilateral or bilateral infiltrates on chest radiograph. Despite several trials, there are no targeted therapies for adult ARDS (12-23) or for PARDS (24-27) beyond supportive care (28,29). Although PARDS management is largely extrapolated from adults, PARDS possesses distinct epidemiology (11,30) and outcomes (31), making application of adult data challenging (30,32-34). While the etiologies of PARDS are similar to adult ARDS, consisting predominantly of pneumonia and non-pulmonary sepsis (35), there has been substantially less investigation into the immune dysregulation in PARDS, and whether or how it differs from adult ARDS.
Innate immunity and neutrophilic lung infiltration are the focus of multiple pre-clinical and translational studies of ARDS, as they are thought to represent very upstream inciting events (4,36). Microbial particles and endogenous damage-associated molecular patterns (DAMPS), which are markers of host cellular injury, activate pulmonary epithelium and macrophages and initiate the inflammatory process (37). Pulmonary macrophage activation promotes neutrophil recruitment to the lung which leads to a host of downstream inflammatory cascades. This inflammatory milieu results in disruption of the alveolar-capillary barrier, causing accumulation of proteinaceous pulmonary edema, with resultant hypoxemia and reduced pulmonary compliance. In the following review, we will address several aspects of the immune dysregulation in adult ARDS and in PARDS (Table 1, Figure 1), including pre-clinical, translational, and clinical evidence to date, with a view towards future therapeutics. We present the following article in accordance with the Narrative Review reporting checklist (available at http://dx.doi.org/10.21037/tp-20-341).
Table 1
Category | Specific components | Proposed therapies |
---|---|---|
DAMPs | Histones | Heparin |
C1 esterase inhibitor | ||
cfDNA/mtDNA | RBC scavenging of mtDNA | |
Innate immunity | NLRP3 inflammasome | Caspase-1 inhibition |
NETosis | PAD4 inhibition | |
PF4-mediated NET stabilization | ||
Pleiotropic anti-inflammatories | Corticosteroids | |
IFN-β | ||
Adaptive immunity | Th17/Treg balance | T cell re-programming |
cffDNA, cell free DNA; DAMPs, damage-associated molecular patterns; IFN, interferon; mtDNA, mitochondrial DNA; PAD4, peptidylarginine deiminase 4; PF4, platelet factor 4; RBC, red blood cell.
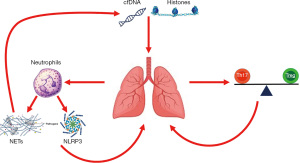
Methods
We performed a semi-systematic review of the literature using PubMed from 1967 to October 2020, with a preference for articles published after 2000. Our aim was consistent with that of a narrative, not a systematic, review. Search terms included every iteration of ARDS and PARDS, including “acute respiratory failure,” paired with the terms “immune,” “innate immun#,” “adaptive immun#,” “damage-associated molecular patterns,” “neutrophils,” “B cells,” and “T cells.” We were interested in summarizing data about the potential druggable targets in the immune dysregulation of ARDS and PARDS, and so prioritized articles which specifically discussed immunomodulation.
Damage-associated molecular patterns
DAMPs have been invoked as major upstream mediators of lung injury. Released after cellular injury in response to an insult, DAMPs are endogenous moieties which are recognized by pattern recognition receptors (PRRs) as damaging. DAMPs include high mobility group box 1 (HMGB1), histones, and cell-free DNA (cfDNA)—primarily mitochondrial DNA (mtDNA) (38). PRRs, including Toll-like receptors (TLRs), nucleotide-binding oligomerization domain-like receptors (NLRs), and the receptor for advanced glycation end-products (RAGE), have evolved alongside pathogens to recognize infectious entities as foreign and mount appropriate responses against them. However, endogenous DAMPs share sufficient structure with some infectious particles that they can activate these same inflammatory pathways via PRR signaling. Several of these molecules are released into the circulation after regulated or unregulated cell death after an inciting insult, exposing normally cytosolic and nuclear proteins to PRRs in the immune system and endothelial surface.
Nuclear proteins such as HMGB1 and histones are pathogenic in ARDS. HMGB1 can propagate inflammation via activating RAGE (39,40), while histones can amplify inflammation via both TLR-dependent and independent mechanisms (41). Histones are implicated in sepsis (42-45), aspiration (46), and trauma-related ARDS (47) in adults. Exogenous histone administration can also induce formation of bactericidal neutrophil extracellular traps (NETs) (45), creating a feedback loop wherein NETosis and tissue death during the initial response to an insult release additional histones into the circulation, which in turn induce further NET formation. A single study in children showed that nucleosomes, the histone/DNA complexes resulting from nuclear chromatin degradation released after cell death, were higher in PARDS non-survivors and correlated with organ failures (48).
The precise mechanisms of histone-induced cytotoxicity are the focus of current investigations. Signaling through TLR2 and TLR4 have been implicated (42,49,50); however, direct binding of histones to the cell surface membrane causing calcium influx and subsequent cell lysis has also been described (47). Given the upstream position of histones and other DAMPs in the propagation of lung injury, they are attractive therapeutic targets. Treatment with low-dose, sub-anticoagulant doses of heparin attenuates the cytotoxicity of histones in pre-clinical models of sepsis. The highly negatively charged heparin mitigated cytotoxicity and improved survival by complexing and inactivating positively charged histones (51). In a separate study, the negatively charged carbohydrate moieties on C1 esterase inhibitor were able to neutralize circulating histones, thereby attenuating histone-induced cytotoxicity and lung injury (52). The availability and existing medical indications for heparin and C1 esterase inhibitor make these attractive options for future studies aimed at mitigating or preventing ARDS.
Histones have also been implicated as causing cytotoxicity via TLR9 (53), which is a sensor for hypomethylated CpG DNA, a distinct type of DAMP. It is possible that histones increase availability and presentation of cfDNA, and so indirectly effect cytotoxicity via TLR9. However, it should be noted that nuclear DNA, the type most likely associated with histones, are not clearly DAMPs. While plasma nuclear DNA levels are associated with degree of shock, organ failure, and inflammatory cytokine levels (54), it is not clear that they are themselves pathogenic. By contrast, methylation of the CpG regions in mtDNA differs from nuclear DNA, rendering mtDNA more similar to bacterial DNA and thus capable of activating TLR9 as a DAMP. In ARDS, mtDNA levels are associated with increased endothelial permeability (55) and lung injury (56). Pre-clinical studies using ex vivo rat lungs demonstrate that targeting mtDNA repair can attenuate pulmonary endothelial permeability (55). Circulating mtDNA has also been associated with mortality in critically ill adults (57,58). Recently, it has been demonstrated in a landmark translational study using mice and humans that under normal homeostatic conditions, red blood cells express TLR9 and clear endogenous mtDNA via direct binding (56). During periods of acute inflammation, this clearance is affected such that levels of circulating mtDNA rises, contributing to lung injury. Loss of TLR9 on red blood cells via knockout decreased mtDNA scavenging and increased lung injury. Harnessing known pathways of mtDNA repair or exploiting existing scavenging pathways may be potential therapeutic avenues for mitigating ARDS after acute inflammatory insults, such as sepsis and trauma.
Innate immunity
Neutrophils and the innate immune system have been implicated in ARDS since its initial description. In two separate trials, elevated plasma interleukin (IL)-6, IL-8, and tumor necrosis factor (TNF)-α, and elevated alveolar IL-6, TNF-α, and IL-1β, were associated with higher tidal volumes and pressure limits (59,60). Furthermore, re-analyses of multiple ARDSNetwork trials have demonstrated the likely presence of two distinct subtypes defined, in part, by inflammatory biomarkers, including IL-6, IL-8, and TNF-receptor 1 (61,62). These studies confirm the relevance of the innate immune system and its cytokines in the pathogenesis of ARDS. Whether these insights translate into therapeutic benefits remains to be seen. However, it is notable that re-analyses of trials show a differential response of positive end-expiratory pressure, fluid, and simvastatin dependent on inflammatory subtype (61-63), suggesting the possibility of predictive enrichment based on innate immune biomarkers in ARDS. The relevance of this paradigm in PARDS has yet to be demonstrated.
Inflammasome
Inflammasomes are intracellular protein complexes activated during infection or stress, including by DAMPs. The best studied is the NLRP3 inflammasome, which activate caspase-1, which in turn activates IL-1β and IL-18 (64). After demonstrating elevated IL-1β and IL-18 in ARDS, investigators assessed the relevance of the inflammasome in a mouse model of ventilator-induced lung injury (VILI) (65). VILI induced elevated inflammasome cytokines, with the alveolar macrophage as the likely origin. Genetic deletion of either IL-18 or caspase-1 conferred resistance to VILI, as did pharmacologic inhibition of IL-18. The relevance of the inflammasome was confirmed in a separate model of lipopolysaccharide (LPS) + VILI, in which genetic deletion of caspase-1 and pharmacologic inhibition of IL-1β protected mice from hypoxemia (66). Deletion of caspase-1 also protected mice subject to intratracheal LPS (67). Finally, pharmacologic IL-1β inhibition has been demonstrated to protect rats from VILI (68).
Importantly, all of the models described above implying benefit to neutralization of the inflammasome or its cytokines were sterile, and did not involve live bacteria. In a mouse pneumonia model using Pseudomonas aeruginosa, inflammasome induction was associated with impaired bacterial clearance and higher mortality (69). Depletion of alveolar macrophages, inhibition of caspase-1, or genetic deletion of IL-1β or IL-18 improved bacterial clearance and mortality, suggesting a therapeutic role for inflammasome inhibition even in an injury model with live bacteria.
A separate investigation investigating the role of Staphylococcus aureus alpha toxin provides some additional nuance to these findings (70). In this mouse pneumonia model, administration of either Staphylococcus or alpha toxin was sufficient to activate the inflammasome, and inflammasome activation prevented bacterial clearance. In this model, inhibition of either or both of the downstream cytokines IL-1β and IL-18 failed to improve bacterial clearance or mortality. However, direct inhibition of the inflammasome itself or inhibition of caspase-1 improved bacterial clearance and mortality. This was due to re-direction of cellular mitochondria away from inflammasomes and towards phagosomes containing bacteria, an effect which was mediated by alpha toxin. In other words, Staphylococcus aureus alpha toxin induces inflammasome activation, drawing mitochondria towards the inflammasome and away from bacteria-containing phagosomes, thereby preventing bacterial clearance. In this model, inflammasome inhibition, but not cytokine inhibition, improved bacterial clearance and outcomes. As pneumonia is the most common cause of ARDS, premature trials of IL-1β or IL-18 inhibition in human ARDS may be doomed to failure, due to an imprecise appreciation of the underlying pathophysiology, despite promising pre-clinical data (71). These studies also highlight the importance of testing therapies in multiple lung injury models, some of which need to include live bacteria, given the prevalence of infection as an inciting etiology in human ARDS.
Neutrophil extracellular traps (NETs)
The neutrophil influx in response to alveolar epithelial and macrophage signaling is proportional to the degree of lung injury (72). NETs are a form of programmed neutrophil cell death regulated by peptidylarginine deiminase 4 (PAD4) citrullination of histones (73-78). NETosis can be initiated by TLR4-dependent signaling on platelets (74), and the resulting extracellular lattices of chromatin and antimicrobial factors capture pathogens, but also can cause pulmonary injury and release additional DAMPs (79). As PAD4 is essential for NETosis, it has emerged as a potential therapeutic target. Preventing histone citrullination improved survival in a mouse abdominal sepsis model (77). However, a more nuanced view of NETs requires an acknowledgement of their beneficial antimicrobial properties. Studies investigating NET stability offer insight into balancing the beneficial and toxic effects of NETs in vivo (80). Platelet factor 4 (PF4), a platelet-associated chemokine, binds, compacts, and stabilizes NETs in the microvasculature, increasing their resistance to DNase I. PF4 increased NET-mediated bacterial capture, reduced the release of NET degradation products, and improved outcomes in murine sepsis (80). These effects were further augmented by a modified monoclonal antibody which further stabilized PF4-NET complexes, supporting a NET-targeted approach to improving inflammatory lung injury.
T cells and adaptive immunity
Inflammatory cells other than neutrophils have also been implicated in ARDS. Most recently, the involvement of CD4+ T-cells have been investigated (81-84). Several Th subsets within the T cell immune system are now well defined, including Th1, Th2, Th17, and regulatory T cells (Tregs). Tregs are a subtype of T cells required for maintaining immune homeostasis and maintaining self-tolerance. A pivotal study showed that CD4+ CD25+ FOXp3+ Tregs were able to resolve experimental lung injury in a murine model (82), consistent with an anti-inflammatory role. Tregs have also been detected in the alveolar fluid of adults with ARDS (82,84), suggesting a potentially modifiable cell-mediated immunotherapy option for ARDS. However, limiting this as an option is a lack of clarity regarding the precise mechanism of Tregs in ameliorating lung injury, as well as a lack of existing therapies.
Th17 cells have also been implicated in ARDS. αβTh17 cells are helper T cells that secrete a distinct subset of cytokines, including IL-17, IL-21, and IL-22 (85). In pre-clinical studies, mice either genetically modified or pharmacologically inhibited IL-17 signaling were protected from experimental lung injury (86), suggesting an antigen-specific adaptive immune dysregulation component to ARDS. IL-17 has been found elevated in the alveolar fluid (86,87) and plasma (87), and correlated with higher pulmonary neutrophilia and with severity of organ failures, suggesting plausibility as a therapeutic target. Under certain inflammatory conditions, Tregs can be reprogrammed into Th17 cells (88). In a single study assessing the ratio of peripheral Th17/Treg cells in ARDS, a higher ratio in favor of Th17 was associated with more organ dysfunction, worse oxygenation, and worse survival (83). Further investigations into shifting the balance towards Tregs may offer a potential therapeutic avenue for ARDS.
Immunomodulation
The earliest attempts at immunomodulation in ARDS, including in the initial report in 1967 (3), were corticosteroids. While Ashbaugh et al. listed corticosteroids under “Therapeutic Trials of Doubtful value,” the anti-inflammatory effects of corticosteroids have long held appeal for the inflammation presumed to drive ARDS. A trial of brief high-dose (120 mg/kg in one day) methylprednisolone did not demonstrate benefit in ARDS (89), but interest was rekindled when a small subsequent trial in persistent (for at least 7 days) ARDS demonstrated possible efficacy of a lower but prolonged dose of methylprednisolone (starting at 2 mg/kg/day with subsequent weaning) (90). A subsequent larger trial failed to replicate these findings, with suggestion of harm if methylprednisolone was initiated >14 days after ARDS onset (14). In both trials, duration of ventilation improved, suggesting a consistent signal for methylprednisolone improving lung function. However, the larger trial demonstrated higher rates of neuromuscular weakness and re-intubations with methylprednisolone, with ultimately no beneficial effect relative to placebo by 60 days. Most recently, an open-label trial of dexamethasone in acute ARDS showed improvement in both mortality and ventilator duration (91).
Overall, the untargeted anti-inflammatory effect of corticosteroids appears to consistently reduce lung inflammation and shorten duration of ventilation in ARDS, with inconsistent effects on mortality. Future investigations should validate these findings, and also clarify issues regarding corticosteroid type, timing, and duration. It is notable that corticosteroid trials have not always improved mortality despite generally improving lung injury. Pre-clinical models of lung injury suggest a hyperinflammatory state which should be improved by pleiotropic immunosuppression by corticosteroids. However, only a minority of deaths in either adult ARDS (92) or PARDS (93) are actually caused by refractory lung injury; multisystem organ failure and neurologic dysfunction contribute to more deaths than hypoxemia. Thus, it is possible that corticosteroids may fail to consistently improve mortality in trials despite improving lung injury because of the disassociation of lung injury from the multiple causes of mortality in ARDS. Alternatively, it is possible that the off-target side effects of corticosteroids, such as neuromuscular weakness and increased risk of secondary infections, counteract any beneficial effects on lung injury. Balancing risks and benefits of untargeted immunomodulators like corticosteroids requires a better mechanistic understanding of ARDS, an appreciation of the causal links between lung inflammation and outcomes, and appropriate trial design.
There are few studies of targeted immunomodulation in ARDS. Cluster of differentiation 73 (CD73) is expressed on endothelium, epithelial cells, and leukocytes. CD73 is anti-inflammatory, prevents vascular leakage (94), and is upregulated by interferon-β (IFN-β) (95). A phase 1-2 trial tested different doses of exogenous IFN-β, and then assessed the effect of the optimal dose on mortality (96). IFN-β was associated with a dramatic reduction in mortality in this pilot. A follow-up multicenter phase 3 trial did not demonstrate any efficacy for IFN-β (97). The authors noted the high (> 50% in both arms) prevalence of corticosteroid use in the trial, with worse outcomes in the IFN-β arm in subjects concurrently receiving corticosteroids. This is relevant, as corticosteroids inhibit interferon signaling (98), and thus may have prevented the anti-inflammatory and vascular stabilizing effects of IFN-β. Given the ubiquitous use of corticosteroids in both adult ARDS (99) and PARDS (100,101), this trial also demonstrates the multiple considerations involved in designing a trial of a targeted immunomodulator.
Future directions
The development of deep immunophenotyping techniques, which allow multiple cell surface markers to be simultaneously detected more efficiently than by traditional flow cytometry. This type of technology fills an important gap in our current understanding between transcriptomics and circulating biomarkers. Better characterization of the specific immune cell populations in both the alveolar space and in the circulation will improve our understanding of the nuanced balance between pro- and anti-inflammatory signaling in health and in ARDS. Immune cells can also be used to identify sub-phenotypes of ARDS, similar to how plasma biomarkers have been proposed for reducing ARDS heterogeneity (62,63,102). Immune-derived sub-phenotypes may be a more informative strategy for predictive enrichment for future immunomodulating therapies in ARDS, as the link between presumed pathophysiology and proposed therapy is more direct. Better characterization of the immune dysregulation of ARDS is essential to disentangling the complexity of ARDS which has plagued our field and contributed to over 50 years of near-universal negative trials.
Acknowledgments
Funding: K23-HL136688; R01-HL148054.
Footnote
Provenance and Peer Review: This article was commissioned by the Guest Editors (Jan Hau Lee, Vijay Srinivasan, and Debbie Long) for the series “Pediatric Critical Care” published in Translational Pediatrics. The article has undergone external peer review.
Reporting Checklist: The author has completed the Narrative Review reporting checklist. Available at http://dx.doi.org/10.21037/tp-20-341
Conflicts of Interest: The author has completed the ICMJE uniform disclosure form (available at http://dx.doi.org/10.21037/tp-20-341). The series “Pediatric Critical Care” was commissioned by the editorial office without any funding or sponsorship. Dr. Yehya’s institution receives grant support from NIH and from Pfizer, both outside of the scope of this work.
Ethical Statement: The author is accountable for all aspects of the work in ensuring that questions related to the accuracy or integrity of any part of the work are appropriately investigated and resolved.
Open Access Statement: This is an Open Access article distributed in accordance with the Creative Commons Attribution-NonCommercial-NoDerivs 4.0 International License (CC BY-NC-ND 4.0), which permits the non-commercial replication and distribution of the article with the strict proviso that no changes or edits are made and the original work is properly cited (including links to both the formal publication through the relevant DOI and the license). See: https://creativecommons.org/licenses/by-nc-nd/4.0/.
References
- Bernard GR, Artigas A, Brigham KL, et al. The American-European Consensus Conference on ARDS. Definitions, mechanisms, relevant outcomes, and clinical trial coordination. Am J Respir Crit Care Med 1994;149:818-24. [Crossref] [PubMed]
- Force ADT, Ranieri VM, Rubenfeld GD, et al. Acute respiratory distress syndrome: the Berlin Definition. JAMA 2012;307:2526-33. [PubMed]
- Ashbaugh DG, Bigelow DB, Petty TL, et al. Acute respiratory distress in adults. Lancet 1967;2:319-23. [Crossref] [PubMed]
- Matthay MA, Ware LB, Zimmerman GA. The acute respiratory distress syndrome. J Clin Invest 2012;122:2731-40. [Crossref] [PubMed]
- Zimmerman JJ, Akhtar SR, Caldwell E, et al. Incidence and outcomes of pediatric acute lung injury. Pediatrics 2009;124:87-95. [Crossref] [PubMed]
- Yehya N, Servaes S, Thomas NJ. Characterizing degree of lung injury in pediatric acute respiratory distress syndrome. Crit Care Med 2015;43:937-46. [Crossref] [PubMed]
- Flori HR, Glidden DV, Rutherford GW, et al. Pediatric acute lung injury: prospective evaluation of risk factors associated with mortality. Am J Respir Crit Care Med 2005;171:995-1001. [Crossref] [PubMed]
- Lopez-Fernandez Y, Azagra AM, de la Oliva P, et al. Pediatric Acute Lung Injury Epidemiology and Natural History study: Incidence and outcome of the acute respiratory distress syndrome in children. Crit Care Med 2012;40:3238-45. [Crossref] [PubMed]
- Khemani RG, Rubin S, Belani S, et al. Pulse oximetry vs. PaO2 metrics in mechanically ventilated children: Berlin definition of ARDS and mortality risk. Intensive Care Med 2015;41:94-102. [Crossref] [PubMed]
- Schouten LR, Veltkamp F, Bos AP, et al. Incidence and Mortality of Acute Respiratory Distress Syndrome in Children: A Systematic Review and Meta-Analysis. Crit Care Med 2016;44:819-29. [Crossref] [PubMed]
- Khemani RG, Smith LS, Zimmerman JJ, et al. Pediatric acute respiratory distress syndrome: definition, incidence, and epidemiology: proceedings from the Pediatric Acute Lung Injury Consensus Conference. Pediatr Crit Care Med 2015;16:S23-40. [Crossref] [PubMed]
- Taylor RW, Zimmerman JL, Dellinger RP, et al. Low-dose inhaled nitric oxide in patients with acute lung injury: a randomized controlled trial. JAMA 2004;291:1603-9. [Crossref] [PubMed]
- Zeiher BG, Artigas A, Vincent JL, et al. Neutrophil elastase inhibition in acute lung injury: results of the STRIVE study. Crit Care Med 2004;32:1695-702. [Crossref] [PubMed]
- Steinberg KP, Hudson LD, Goodman RB, et al. Efficacy and safety of corticosteroids for persistent acute respiratory distress syndrome. N Engl J Med 2006;354:1671-84. [Crossref] [PubMed]
- National Heart L. Randomized, placebo-controlled clinical trial of an aerosolized beta(2)-agonist for treatment of acute lung injury. Am J Respir Crit Care Med 2011;184:561-8. [Crossref] [PubMed]
- Gao Smith F, Perkins GD, Gates S, et al. Effect of intravenous beta-2 agonist treatment on clinical outcomes in acute respiratory distress syndrome (BALTI-2): a multicentre, randomised controlled trial. Lancet 2012;379:229-35. [Crossref] [PubMed]
- McAuley DF, Laffey JG, O'Kane CM, et al. Simvastatin in the acute respiratory distress syndrome. N Engl J Med 2014;371:1695-703. [Crossref] [PubMed]
- National Heart L. Rosuvastatin for sepsis-associated acute respiratory distress syndrome. N Engl J Med 2014;370:2191-200. [Crossref] [PubMed]
- Willson DF, Truwit JD, Conaway MR, et al. The Adult Calfactant in Acute Respiratory Distress Syndrome Trial. Chest 2015;148:356-64. [Crossref] [PubMed]
- . Ketoconazole for early treatment of acute lung injury and acute respiratory distress syndrome: a randomized controlled trial. The ARDS Network. JAMA 2000;283:1995-2002. [Crossref] [PubMed]
- Anzueto A, Baughman RP, Guntupalli KK, et al. Aerosolized surfactant in adults with sepsis-induced acute respiratory distress syndrome. Exosurf Acute Respiratory Distress Syndrome Sepsis Study Group. N Engl J Med 1996;334:1417-21. [Crossref] [PubMed]
- Bone RC, Slotman G, Maunder R, et al. Randomized double-blind, multicenter study of prostaglandin E1 in patients with the adult respiratory distress syndrome. Prostaglandin E1 Study Group. Chest 1989;96:114-9. [Crossref] [PubMed]
- Spragg RG, Lewis JF, Walmrath HD, et al. Effect of recombinant surfactant protein C-based surfactant on the acute respiratory distress syndrome. N Engl J Med 2004;351:884-92. [Crossref] [PubMed]
- Willson DF, Thomas NJ, Markovitz BP, et al. Effect of exogenous surfactant (calfactant) in pediatric acute lung injury: a randomized controlled trial. JAMA 2005;293:470-6. [Crossref] [PubMed]
- Thomas NJ, Guardia CG, Moya FR, et al. A pilot, randomized, controlled clinical trial of lucinactant, a peptide-containing synthetic surfactant, in infants with acute hypoxemic respiratory failure. Pediatr Crit Care Med 2012;13:646-53. [Crossref] [PubMed]
- Willson DF, Thomas NJ, Tamburro R, et al. Pediatric calfactant in acute respiratory distress syndrome trial. Pediatr Crit Care Med 2013;14:657-65. [Crossref] [PubMed]
- Drago BB, Kimura D, Rovnaghi CR, et al. Double-blind, placebo-controlled pilot randomized trial of methylprednisolone infusion in pediatric acute respiratory distress syndrome. Pediatr Crit Care Med 2015;16:e74-81. [Crossref] [PubMed]
- Acute Respiratory Distress Syndrome N, Brower RG, Matthay MA, et al. Ventilation with lower tidal volumes as compared with traditional tidal volumes for acute lung injury and the acute respiratory distress syndrome. N Engl J Med 2000;342:1301-8. [Crossref] [PubMed]
- National Heart L. Comparison of two fluid-management strategies in acute lung injury. N Engl J Med 2006;354:2564-75. [Crossref] [PubMed]
- Yehya N, Keim G, Thomas NJ. Subtypes of pediatric acute respiratory distress syndrome have different predictors of mortality. Intensive Care Med 2018;44:1230-9. [Crossref] [PubMed]
- Quasney MW, Lopez-Fernandez YM, Santschi M, et al. The outcomes of children with pediatric acute respiratory distress syndrome: proceedings from the Pediatric Acute Lung Injury Consensus Conference. Pediatr Crit Care Med 2015;16:S118-31. [Crossref] [PubMed]
- De Luca D, Piastra M, Chidini G, et al. The use of the Berlin definition for acute respiratory distress syndrome during infancy and early childhood: multicenter evaluation and expert consensus. Intensive Care Med 2013;39:2083-91. [Crossref] [PubMed]
- de Jager P, Burgerhof JG, van Heerde M, et al. Tidal volume and mortality in mechanically ventilated children: a systematic review and meta-analysis of observational studies*. Crit Care Med 2014;42:2461-72. [Crossref] [PubMed]
- Yehya N, Thomas NJ. Disassociating Lung Mechanics and Oxygenation in Pediatric Acute Respiratory Distress Syndrome. Crit Care Med 2017;45:1232-9. [Crossref] [PubMed]
- Khemani RG, Smith L, Lopez-Fernandez YM, et al. Paediatric acute respiratory distress syndrome incidence and epidemiology (PARDIE): an international, observational study. Lancet Respir Med 2019;7:115-28. [Crossref] [PubMed]
- Ware LB, Matthay MA. The acute respiratory distress syndrome. N Engl J Med 2000;342:1334-49. [Crossref] [PubMed]
- Opitz B, van Laak V, Eitel J, et al. Innate immune recognition in infectious and noninfectious diseases of the lung. Am J Respir Crit Care Med 2010;181:1294-309. [Crossref] [PubMed]
- Xiang M, Fan J. Pattern recognition receptor-dependent mechanisms of acute lung injury. Mol Med 2010;16:69-82. [Crossref] [PubMed]
- Achouiti A, van der Meer AJ, Florquin S, et al. High-mobility group box 1 and the receptor for advanced glycation end products contribute to lung injury during Staphylococcus aureus pneumonia. Crit Care 2013;17:R296. [Crossref] [PubMed]
- Qing DY, Conegliano D, Shashaty MG, et al. Red blood cells induce necroptosis of lung endothelial cells and increase susceptibility to lung inflammation. Am J Respir Crit Care Med 2014;190:1243-54. [Crossref] [PubMed]
- Marsman G, Zeerleder S, Luken BM. Extracellular histones, cell-free DNA, or nucleosomes: differences in immunostimulation. Cell Death Dis 2016;7:e2518 [Crossref] [PubMed]
- Xu J, Zhang X, Pelayo R, et al. Extracellular histones are major mediators of death in sepsis. Nat Med 2009;15:1318-21. [Crossref] [PubMed]
- Ekaney ML, Otto GP, Sossdorf M, et al. Impact of plasma histones in human sepsis and their contribution to cellular injury and inflammation. Crit Care 2014;18:543. [Crossref] [PubMed]
- Alhamdi Y, Abrams ST, Cheng Z, et al. Circulating Histones Are Major Mediators of Cardiac Injury in Patients With Sepsis. Crit Care Med 2015;43:2094-103. [Crossref] [PubMed]
- Raffray L, Douchet I, Augusto JF, et al. Septic shock sera containing circulating histones induce dendritic cell-regulated necrosis in fatal septic shock patients. Crit Care Med 2015;43:e107-16. [Crossref] [PubMed]
- Zhang Y, Wen Z, Guan L, et al. Extracellular histones play an inflammatory role in acid aspiration-induced acute respiratory distress syndrome. Anesthesiology 2015;122:127-39. [Crossref] [PubMed]
- Abrams ST, Zhang N, Manson J, et al. Circulating histones are mediators of trauma-associated lung injury. Am J Respir Crit Care Med 2013;187:160-9. [Crossref] [PubMed]
- Yehya N, Thomas NJ, Margulies SS. Circulating nucleosomes are associated with mortality in pediatric acute respiratory distress syndrome. Am J Physiol Lung Cell Mol Physiol 2016;310:L1177-84. [Crossref] [PubMed]
- Xu J, Zhang X, Monestier M, et al. Extracellular histones are mediators of death through TLR2 and TLR4 in mouse fatal liver injury. J Immunol 2011;187:2626-31. [Crossref] [PubMed]
- Allam R, Scherbaum CR, Darisipudi MN, et al. Histones from dying renal cells aggravate kidney injury via TLR2 and TLR4. J Am Soc Nephrol 2012;23:1375-88. [Crossref] [PubMed]
- Wildhagen KC, Garcia de Frutos P, Reutelingsperger CP, et al. Nonanticoagulant heparin prevents histone-mediated cytotoxicity in vitro and improves survival in sepsis. Blood 2014;123:1098-101. [Crossref] [PubMed]
- Wygrecka M, Kosanovic D, Wujak L, et al. Antihistone Properties of C1 Esterase Inhibitor Protect against Lung Injury. Am J Respir Crit Care Med 2017;196:186-99. [Crossref] [PubMed]
- Huang H, Evankovich J, Yan W, et al. Endogenous histones function as alarmins in sterile inflammatory liver injury through Toll-like receptor 9 in mice. Hepatology 2011;54:999-1008. [Crossref] [PubMed]
- Timmermans K, Kox M, Scheffer GJ, et al. Plasma Nuclear and Mitochondrial DNA Levels, and Markers of Inflammation, Shock, and Organ Damage in Patients with Septic Shock. Shock 2016;45:607-12. [Crossref] [PubMed]
- Chouteau JM, Obiako B, Gorodnya OM, et al. Mitochondrial DNA integrity may be a determinant of endothelial barrier properties in oxidant-challenged rat lungs. Am J Physiol Lung Cell Mol Physiol 2011;301:L892-8. [Crossref] [PubMed]
- Hotz MJ, Qing D, Shashaty MGS, et al. Red Blood Cells Homeostatically Bind Mitochondrial DNA through TLR9 to Maintain Quiescence and to Prevent Lung Injury. Am J Respir Crit Care Med 2018;197:470-80. [Crossref] [PubMed]
- Nakahira K, Kyung SY, Rogers AJ, et al. Circulating mitochondrial DNA in patients in the ICU as a marker of mortality: derivation and validation. PLoS Med 2013;10:e1001577-discussion e1001577. [Crossref] [PubMed]
- Simmons JD, Lee YL, Mulekar S, et al. Elevated levels of plasma mitochondrial DNA DAMPs are linked to clinical outcome in severely injured human subjects. Ann Surg 2013;258:591-6; discussion 596-8. [Crossref] [PubMed]
- Ranieri VM, Suter PM, Tortorella C, et al. Effect of mechanical ventilation on inflammatory mediators in patients with acute respiratory distress syndrome: a randomized controlled trial. JAMA 1999;282:54-61. [Crossref] [PubMed]
- Parsons PE, Eisner MD, Thompson BT, et al. Lower tidal volume ventilation and plasma cytokine markers of inflammation in patients with acute lung injury. Crit Care Med 2005;33:1-6; discussion 230-2. [Crossref] [PubMed]
- Calfee CS, Delucchi K, Parsons PE, et al. Subphenotypes in acute respiratory distress syndrome: latent class analysis of data from two randomised controlled trials. Lancet Respir Med 2014;2:611-20. [Crossref] [PubMed]
- Famous KR, Delucchi K, Ware LB, et al. Acute Respiratory Distress Syndrome Subphenotypes Respond Differently to Randomized Fluid Management Strategy. Am J Respir Crit Care Med 2017;195:331-8. [Crossref] [PubMed]
- Calfee CS, Delucchi KL, Sinha P, et al. Acute respiratory distress syndrome subphenotypes and differential response to simvastatin: secondary analysis of a randomised controlled trial. Lancet Respir Med 2018;6:691-8. [Crossref] [PubMed]
- Schroder K, Tschopp J. The inflammasomes. Cell 2010;140:821-32. [Crossref] [PubMed]
- Dolinay T, Kim YS, Howrylak J, et al. Inflammasome-regulated cytokines are critical mediators of acute lung injury. Am J Respir Crit Care Med 2012;185:1225-34. [Crossref] [PubMed]
- Jones HD, Crother TR, Gonzalez-Villalobos RA, et al. The NLRP3 inflammasome is required for the development of hypoxemia in LPS/mechanical ventilation acute lung injury. Am J Respir Cell Mol Biol 2014;50:270-80. [PubMed]
- Grailer JJ, Canning BA, Kalbitz M, et al. Critical role for the NLRP3 inflammasome during acute lung injury. J Immunol 2014;192:5974-83. [Crossref] [PubMed]
- Frank JA, Pittet JF, Wray C, et al. Protection from experimental ventilator-induced acute lung injury by IL-1 receptor blockade. Thorax 2008;63:147-53. [PubMed]
- Cohen TS, Prince AS. Activation of inflammasome signaling mediates pathology of acute P. aeruginosa pneumonia. J Clin Invest 2013;123:1630-7. [Crossref] [PubMed]
- Cohen TS, Boland ML, Boland BB, et al. S. aureus Evades Macrophage Killing through NLRP3-Dependent Effects on Mitochondrial Trafficking. Cell Rep 2018;22:2431-41. [Crossref] [PubMed]
- Vanden Berghe T, Demon D, Bogaert P, et al. Simultaneous targeting of IL-1 and IL-18 is required for protection against inflammatory and septic shock. Am J Respir Crit Care Med 2014;189:282-91. [Crossref] [PubMed]
- Williams AE, Chambers RC. The mercurial nature of neutrophils: still an enigma in ARDS? Am J Physiol Lung Cell Mol Physiol 2014;306:L217-30. [Crossref] [PubMed]
- Brinkmann V, Reichard U, Goosmann C, et al. Neutrophil extracellular traps kill bacteria. Science 2004;303:1532-5. [Crossref] [PubMed]
- Clark SR, Ma AC, Tavener SA, et al. Platelet TLR4 activates neutrophil extracellular traps to ensnare bacteria in septic blood. Nat Med 2007;13:463-9. [Crossref] [PubMed]
- Demers M, Wagner DD. NETosis: a new factor in tumor progression and cancer-associated thrombosis. Semin Thromb Hemost 2014;40:277-83. [Crossref] [PubMed]
- Hirose T, Hamaguchi S, Matsumoto N, et al. Presence of neutrophil extracellular traps and citrullinated histone H3 in the bloodstream of critically ill patients. PLoS One 2014;9:e111755 [Crossref] [PubMed]
- Li Y, Liu Z, Liu B, et al. Citrullinated histone H3: a novel target for the treatment of sepsis. Surgery 2014;156:229-34. [Crossref] [PubMed]
- Li P, Li M, Lindberg MR, et al. PAD4 is essential for antibacterial innate immunity mediated by neutrophil extracellular traps. J Exp Med 2010;207:1853-62. [Crossref] [PubMed]
- Hudock KM, Collins MS, Imbrogno M, et al. Neutrophil extracellular traps activate IL-8 and IL-1 expression in human bronchial epithelia. Am J Physiol Lung Cell Mol Physiol 2020;319:L137-47. [Crossref] [PubMed]
- Gollomp K, Sarkar A, Harikumar S, et al. Fc-modified HIT-like monoclonal antibody as a novel treatment for sepsis. Blood 2020;135:743-54. [Crossref] [PubMed]
- Nakajima T, Suarez CJ, Lin KW, et al. T cell pathways involving CTLA4 contribute to a model of acute lung injury. J Immunol 2010;184:5835-41. [Crossref] [PubMed]
- D'Alessio FR, Tsushima K, Aggarwal NR, et al. CD4+CD25+Foxp3+ Tregs resolve experimental lung injury in mice and are present in humans with acute lung injury. J Clin Invest 2009;119:2898-913. [Crossref] [PubMed]
- Yu ZX, Ji MS, Yan J, et al. The ratio of Th17/Treg cells as a risk indicator in early acute respiratory distress syndrome. Crit Care 2015;19:82. [Crossref] [PubMed]
- Adamzik M, Broll J, Steinmann J, et al. An increased alveolar CD4 + CD25 + Foxp3 + T-regulatory cell ratio in acute respiratory distress syndrome is associated with increased 30-day mortality. Intensive Care Med 2013;39:1743-51. [Crossref] [PubMed]
- Gaffen SL. Structure and signalling in the IL-17 receptor family. Nat Rev Immunol 2009;9:556-67. [Crossref] [PubMed]
- Li JT, Melton AC, Su G, et al. Unexpected Role for Adaptive alphabetaTh17 Cells in Acute Respiratory Distress Syndrome. J Immunol 2015;195:87-95. [Crossref] [PubMed]
- Mikacenic C, Hansen EE, Radella F, et al. Interleukin-17A Is Associated With Alveolar Inflammation and Poor Outcomes in Acute Respiratory Distress Syndrome. Crit Care Med 2016;44:496-502. [Crossref] [PubMed]
- Barbi J, Pardoll D, Pan F. Metabolic control of the Treg/Th17 axis. Immunol Rev 2013;252:52-77. [Crossref] [PubMed]
- Bernard GR, Luce JM, Sprung CL, et al. High-dose corticosteroids in patients with the adult respiratory distress syndrome. N Engl J Med 1987;317:1565-70. [Crossref] [PubMed]
- Meduri GU, Headley AS, Golden E, et al. Effect of prolonged methylprednisolone therapy in unresolving acute respiratory distress syndrome: a randomized controlled trial. JAMA 1998;280:159-65. [Crossref] [PubMed]
- Villar J, Ferrando C, Martinez D, et al. Dexamethasone treatment for the acute respiratory distress syndrome: a multicentre, randomised controlled trial. Lancet Respir Med 2020;8:267-76. [Crossref] [PubMed]
- Stapleton RD, Wang BM, Hudson LD, et al. Causes and timing of death in patients with ARDS. Chest 2005;128:525-32. [Crossref] [PubMed]
- Dowell JC, Parvathaneni K, Thomas NJ, et al. Epidemiology of Cause of Death in Pediatric Acute Respiratory Distress Syndrome. Crit Care Med 2018;46:1811-9. [Crossref] [PubMed]
- Colgan SP, Eltzschig HK, Eckle T, et al. Physiological roles for ecto-5'-nucleotidase (CD73). Purinergic Signal 2006;2:351-60. [Crossref] [PubMed]
- Kiss J, Yegutkin GG, Koskinen K, et al. IFN-beta protects from vascular leakage via up-regulation of CD73. Eur J Immunol 2007;37:3334-8. [Crossref] [PubMed]
- Bellingan G, Maksimow M, Howell DC, et al. The effect of intravenous interferon-beta-1a (FP-1201) on lung CD73 expression and on acute respiratory distress syndrome mortality: an open-label study. Lancet Respir Med 2014;2:98-107. [Crossref] [PubMed]
- Ranieri VM, Pettila V, Karvonen MK, et al. Effect of Intravenous Interferon beta-1a on Death and Days Free From Mechanical Ventilation Among Patients With Moderate to Severe Acute Respiratory Distress Syndrome: A Randomized Clinical Trial. JAMA 2020; Epub ahead of print. [Crossref] [PubMed]
- Flammer JR, Dobrovolna J, Kennedy MA, et al. The type I interferon signaling pathway is a target for glucocorticoid inhibition. Mol Cell Biol 2010;30:4564-74. [Crossref] [PubMed]
- Bellani G, Laffey JG, Pham T, et al. Epidemiology, Patterns of Care, and Mortality for Patients With Acute Respiratory Distress Syndrome in Intensive Care Units in 50 Countries. JAMA 2016;315:788-800. [Crossref] [PubMed]
- Yehya N, Servaes S, Thomas NJ, et al. Corticosteroid exposure in pediatric acute respiratory distress syndrome. Intensive Care Med 2015;41:1658-66. [Crossref] [PubMed]
- Rowan CM, Klein MJ, Hsing DD, et al. Early Use of Adjunctive Therapies for Pediatric Acute Respiratory Distress Syndrome: A PARDIE Study. Am J Respir Crit Care Med 2020;201:1389-97. [Crossref] [PubMed]
- Calfee CS, Janz DR, Bernard GR, et al. Distinct molecular phenotypes of direct vs indirect ARDS in single-center and multicenter studies. Chest 2015;147:1539-48. [Crossref] [PubMed]