Translational development of difluoromethylornithine (DFMO) for the treatment of neuroblastoma
Editor’s note:
Cancer has been called a metabolic disease for some time. To address the significance of metabolic alterations in cancer, this column on “Metabolism of Childhood Cancer” is launched with Dr. Dinesh Rakheja (Departments of Pathology and Pediatrics, University of Texas Southwestern Medical Center) serving as the column editor. More featured articles are expected to be published in the column.
Introduction
Cancer remains the leading cause of death by disease in children, and neuroblastoma contributes substantially to this mortality. While intensified conventional treatments using high-dose chemotherapy with stem cell support, radiotherapy and immunotherapy have reduced mortality (1), overall survival remains at less than 50% and comes at the cost of significant life-long morbidities (2). Despite having entered the era of precision medicine few new drug targets have been identified for neuroblastoma (3). Genomic studies of hundreds of tumors have shown that the most common event driving aggressive neuroblastoma is deregulation of MYC family genes, as amplification of the MYCN oncogene (4) or deregulation of MYC signaling (5) occurs in the majority of high-risk tumors. That MYC genes are bona fide drivers of neuroblastoma has been confirmed in the TH-MYCN transgenic mouse model, in which targeted overexpression of MYCN to the murine neural crest (the tissue of origin for neuroblastoma) leads to a lethal tumor that recapitulates the hallmark features of human neuroblastoma (6). Still, despite 30 years of study no MYC-targeted therapy has reached the clinic.
Direct pharmacologic inhibition of Myc proteins themselves has proven difficult, yet many of the cancer activities promoted by Myc require abundant polyamines. Polyamines are essential cationic chaperones that support many Myc functions, and the genes involved in generating polyamines such as ornithine decarboxylase (Odc) are coordinately regulated to promote polyamine sufficiency in MYC-driven cancers like neuroblastoma (7). This has led to the hypothesis that polyamine metabolism provides a druggable signaling module downstream of Myc, and indeed drugs such as difluoromethylornithine (DFMO; Eflornithine) that inhibit Odc activity can antagonize polyamine synthesis, deplete tumors of polyamines, and inhibit the growth of MYC-deregulated tumors (8,9). Effective pre-emptive blockade of polyamine synthesis can prevent neuroblastoma formation in the transgenic TH-MYCN model supporting that polyamines are indeed required for the full oncogenic potential of activated MYC genes. Similar findings from additional models of cancer initiation has led to the study of DFMO as a chemopreventive agent in numerous clinical settings (10). More importantly, however, is the demonstration that MYC-driven tumors appear to remain dependent on polyamines. In both mouse and human neuroblastoma models the depletion of polyamines from progressing tumors renders them substantially more sensitive to chemotherapy and contributes to durable tumor regression (8).
Extensive pre-clinical data credentialing polyamine depletion as a therapeutic strategy in neuroblastoma have led to multiple early phase clinical trials testing DFMO in children. These studies encompass a range of DFMO doses and clinical settings including treatment of tumors that have relapsed after high-risk therapy, or treatment initiated at the completion of therapy at a time of potential minimal residual disease. To fully leverage the clinical activity of DFMO-based therapies it is imperative that a more detailed understanding of the mechanisms by which polyamine depletion contributes to cancer cell killing be developed. In this review we first discuss the putative cancer cell-directed mechanisms of polyamine depletion activity. Identifying the key mechanism linked to activity provides an opportunity to develop biomarkers predictive of treatment response that can then be used prospectively. Second, we review available evidence that polyamine depletion alters the tumor microenvironment to change it from one that is tumor-permissive through repression of immune system effectors, to one that promotes anti-tumor immunity. This is an important consideration given that an immunotherapy regimen consisting of anti-GD2 targeted antibody with GM-CSF and IL-2 has been shown to improve survival in neuroblastoma (11), and begs the question of whether DFMO exposures that target the tumor microenvironment (and immune effectors) could cooperate with immunotherapy to improve outcomes. We will outline current thinking regarding these putative cancer cell-intrinsic and -extrinsic activities, approaches to address them using pre-clinical models, and the relevance that such findings may have for subsequent drug development efforts focused on DFMO use for neuroblastoma.
Connecting MYC oncogenes, polyamines and tumor progression
Integrative genomic studies including whole genome sequencing have identified a limited number of driver genes for neuroblastoma, including MYCN (4), ALK (12,13), ATRX (14) and ARID1A and ARID1B (15). Of these, MYC genes are by far the most prevalent, with deregulation of MYCN by genomic amplification occurring in ~40% of high-risk tumors. MYCN amplification independently correlates with advanced stage disease and poor outcome and is used worldwide to identify higher-risk patients for more aggressive treatments (4,16). In high-risk tumors without MYCN amplification, gene expression analyses identify MYCN (17) or MYC (5,18) gene signatures indicating that augmented “MYC-signaling” may be essential for aggressive tumor behavior. MYC genes are transcription factors that regulate up to 15% of all human genes (19) yet those necessary for tumorigenesis remain elusive. Myc redirects metabolism and orchestrates enhanced synthesis of DNA, RNA, protein, lipid and polyamine precursors to provide for the energy and biomass needs of proliferating cells. Polyamines themselves feed forward to support Myc functions through ionic and covalent interactions, specifically promoting protein translation and ribogenesis (20). Reduced polyamines are seen in non-proliferating cells, while elevated polyamines accompany both normal and oncogenic proliferation (21,22). The intracellular polyamines (putrescine, spermidine, and spermine) are maintained through highly regulated synthetic, catabolic, uptake and export pathways (Figure 1) (23). The Odc enzyme, encoded by ODC1, decarboxylates ornithine from the urea cycle to create putrescine, from which the higher order polyamines are synthesized. The activities of Odc and the enzyme S-adenosylmethionine decarboxylase (Amd, encoded by the AMD1 gene) are rate-limiting in polyamine biosynthesis, and both are highly regulated with the shortest half-lives (10-30 minutes) of any mammalian enzyme. In addition, Odc is degraded through a process regulated by Odc antizymes (24), while antizyme inhibitors compete to neutralize these (25). Such multiple levels of hierarchical control underscores the importance of tightly regulating Odc activity and polyamine homeostasis to ensure cellular fitness.
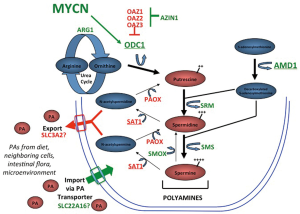
In neuroblastomas, polyamine metabolism is coordinately deregulated to drive polyamine pool expansion (8). Expression of ODC1 is significantly higher and expression of its negative-regulator OAZ2 lower in essentially all high-risk tumors, coordinately enhancing Odc activity and polyamine synthesis. Survival for patients with high tumor ODC1 expression is inferior to those with low ODC1 even when stratified by MYCN status, suggesting ODC1 is not merely a surrogate for MYCN amplification (8). Moreover, the expression of all polyamine synthetic enzymes are upregulated while catabolic enzymes are downregulated in tumors with MYCN amplification, consistent with the heightened MYC signaling in this subset (8). Indeed, in a study of over 650 tumors, high expression of every polyamine synthetic gene (ODC1, AMD1, SRM, SMS, AZIN1), and low expression of every catabolic gene (SAT1, PAOX, OAZ1, OAZ2, OAZ3), correlated with inferior outcome. In multivariable analyses, most of these polyamine genes retain independent prognostic significance when adjusted for patient age, tumor stage and MYCN gene status. Finally, it has been shown that the ODC1 gene, which maps within 5 Mb of MYCN on the short arm of chromosome 2, is co-amplified with MYCN in 15-20% of MYCN amplified tumors, or ~8% of all high-risk tumors (8,15). ODC1 is markedly overexpressed in such tumors, establishing the first example of co-amplification of an oncogenic transcription factor (MYCN) with its oncogenic target gene (ODC1). A similar oncogene-target gene co-amplification event has since been identified in lung carcinoma with PRKC1 and SOX2 driving Hedgehog signalling (26). While the clinical significance of MYCN-ODC1 co-amplification has not been clarified, this subset is predicted to have the highest polyamine metabolic flux and potential dependency.
A functional role for polyamines in supporting neuroblastoma progression has been obtained using complementary pre-clinical mouse models. DFMO is an irreversible inhibitor of Odc and is FDA-approved for the treatment of Trypanosomic encephalitis. It has also been studied as an anti-cancer agent based on its selective Odc inactivation resulting in the reduction of cellular polyamines. The contribution of Odc activity to MYC-induced oncogenesis was first demonstrated using the Eμ-MYC murine lymphoma model in which Odc inhibition using DFMO (or genetic ODC1 deletion) leads to inhibition of lymphomagenesis. This work was the first to suggest a synthetic-lethal interaction between cancer cells with deregulated Myc and polyamine deprivation (9). A similar Myc-dependence on Odc activity and polyamine sufficiency has since been shown using the transgenic TH-MYCN neuroblastoma model (8,27). TH-MYCN mice develop peripheral neural tumors comparable to human neuroblastoma at the genetic (28,29), histologic (30) and ultrastructural level (6). The model is highly aggressive with complete lethal tumor penetrance in transgene homozygous mice (31). Importantly, both polyamine gene expression and metabolite studies confirm this mouse model recapitulates the tumoral polyamine expansion seen in human tumors. Treatment of TH-MYCN homozygous mice prior to the time of tumor onset with DFMO extends survival but all animals eventually die of tumor progression. SAM486 (an Amd inhibitor) is more potent, extending survival while also reducing tumor penetrance. Remarkably, inhibition of both rate-limiting enzymes in polyamine synthesis via the combination of DFMO and SAM486 protects 40% of mice from developing neuroblastoma at all. These data strongly support that polyamine sufficiency is required downstream of MYCN in tumor initiation (8).
A more clinically relevant question is whether established tumors remain dependent on polyamines. It has been shown that the addition of DFMO to chemotherapy extends survival of TH-MYCN mice treated at the time of tumor progression (8). Similar beneficial activity is seen using xenograft models of neuroblastomas with diverse genomic lesions that correlate with poor prognosis, such as MYCN amplification (16), ALK mutation (32), and TP53 mutation with multidrug resistance (33). Collectively, these complementary models have been used to test numerous polyamine antagonist regimens in combination with diverse classes of cytotoxics used to treat human neuroblastoma. Such broad pre-clinical data are critical for prioritizing agents for clinical investigation since agents with more limited pre-clinical testing often fail to demonstrate meaningful activity in human trials (34). To date, the most effective combination for treating established tumors includes both DFMO and celecoxib. Celecoxib was tested since cyclo-oxygenase inhibitors can induce Sat1 activity leading to increased acetylation and export of polyamines from cells (Figure 1). This is proposed to offset the compensatory increase in polyamine uptake that follows DFMO-mediated depletion of intracellular polyamines. However, cyclo-oxygenase enzymes play pleiotropic roles in cancer, including anti-inflammatory roles, and the synergistic activity in these models has not been conclusively linked to polyamine metabolism. Still, a strong clinical signal for synergistic activity between DFMO and cyclo-oxygenase inhibitors, in this case sulindac, has already been demonstrated in a large randomized, placebo-controlled trial in patients at risk for recurrence of colorectal adenomas (35), another tumor classically linked to MYC hyperactivity. DFMO and sulindac reduced the overall incidence of adenoma recurrence by >70%, and the incidence of multiple or advanced adenoma by >90% in the absence of clinically significant toxicity, an important consideration in the chemoprevention setting.
Potential neuroblast intrinsic anti-tumor activities of polyamine deprivation
Short-term in vitro treatment of neuroblastoma cell lines with DFMO at concentrations >1,000 µM leads to proliferative arrest, and at concentrations from 150-500 µM to reduced colony formation capacity (8,36). The polyamine synthesis arrest from DFMO-mediated Odc inhibition leads to a measurable compensatory increase in polyamine uptake from the environment through selective transporters. A large proportion of DFMO’s inhibitory effects can be rescued by the provision of supplemental polyamines to the culture media, or exacerbated by concomitant exposure to a polyamine transporter inhibitor (37), supporting that these activities are on target and mediated through depletion of polyamines from the tumor cells themselves. Moreover, the degree of inhibition using these read-outs parallels the MYC-signal or polyamine-signal as defined using gene expression signatures across diverse neuroblastoma cell lines (8), supporting these cancer cell-intrinsic activities are linked to polyamine metabolism. Current evidence supports two principal candidate mechanisms for the cell-intrinsic activities of DFMO-based therapies: (I) inhibition of protein translation that prevents Myc from driving protein synthesis sufficient to meet the increased biomass needs of proliferating tumor cells; and (II) depletion of thymidine pools that results from Amd1 hyperactivity in response to reduced intracellular polyamines.
The most conserved set of Myc and MycN target genes function in ribosome biogenesis and protein metabolism to support cell proliferation (19,38). Myc genes act at a signaling node linking the cell cycle machinery to such pathways to ensure daughter cells have increased their biomass prior to cytokinesis. Myc drives a dramatic increase in protein synthesis capacity (39) that is evolutionarily conserved and critical to Myc oncogenesis. Inhibition of protein synthesis capacity, whether through targeted deletion of ribosome genes (40) or pharmacologic inhibition of protein synthesis regulators (41), has synthetic-lethal consequences in Myc-driven cancer models. As discussed, polyamine genes also constitute a core Myc expression program and support protein metabolism (42). Polyamines are required for activation of eIF5A (Figure 2A), an important protein translation factor and the only mammalian protein activated by hypusination, a post-translational modification requiring the polyamine spermidine (47). Intriguingly with respect to neuroblastoma, this polyamine-modified translation initiation factor is essential for neuronal growth and survival (48). Polyamine depletion not only leads to loss of active hypusinated-eIF5A but also impedes protein translation through eIF5A-independent pathways by modulating PERK, eIF2 and 4EBP1, providing further links between the regulation of protein translation by Myc and by polyamines (41,49). Finally, profound polyamine depletion that is experimentally induced by hyperactivation of Sat1 leads to a complete arrest of protein translation in mammalian cells, further supporting this mechanism of activity (50). For these reasons, characterizing the effect of various polyamine depletion regimens on the “Myc-polyamine-protein translation axis”, and correlating this with anti-tumor activity, may provide candidate biomarkers to predict therapeutic activity.
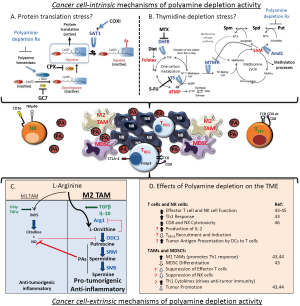
A more recent model hypothesizes that polyamine depletion leads to anti-tumor activity through concomitant depletion of thymidine pools (51). Unbiased metabolic profiling of human colon cancer cells and tumors from mouse models of colon cancer showed DFMO exposure depletes folate-dependent metabolites, including S-adenosylmethionine (SAM), thymidine pools and pathway intermediates (51). This is a consequence of the metabolic link between polyamine synthetic pathways and tetrahydrofolate (THF)-dependent one-carbon transfer reactions, as both require SAM (Figure 2B). Odc inhibition by DFMO reduces putrescine, causing a compensatory hyperactivation of Amd1, which has also been verified in neuroblastoma cells (36). This futile Amd1 cycling depletes SAM, an allosteric inhibitor of MTHFR, driving dTMP precursors toward methionine synthesis and SAM replacement, and away from dTMP synthetic pathways. Depletion of thymidine, in turn, leads to apoptosis due to uridine misincorporation into DNA. Of interest, this cell death mechanism mimics that induced by 5-fluorouracil chemotherapy, commonly used to treat colon cancer, as this agent inhibits thymidylate synthase (TS) to deplete thymidine. Importantly, repletion of DFMO treated colon cancer cells with thymidine rescued cell survival despite persistent polyamine depletion. This again provides an opportunity to correlate the relative impact of various polyamine depletion therapeutics on cell survival and thymidine and folate pools (52). If thymidine depletion were the major mechanism of polyamine depletion activity, one would predict that combining DFMO with SAM486 (an Amd1 inhibitor) might be antagonistic in treating tumors since SAM486 would inhibit the Amd1 hyperactivation that leads to thymidine depletion.
Such basic mechanistic studies may guide how polyamine depletion regimens can be optimized to promote tumor cell stress. If a specific drug effect is credentialed as a dominant contributor to anti-tumor activity then appropriate pharmacodynamic studies can be designed. Drug exposures can be adapted to maximize the desirable biological activity rather than used at a maximal tolerated dose as has been typically done for cancer therapeutics. This may also provide additional opportunities for therapeutic synergy through rational drug combinations (Figure 2A,B). For example, the activation of the essential translation factor eIF5A requires hypusination (spermidine conjugation), a unique protein modification which requires two enzymatic steps catalysed by the deoxyhypusine synthase (DHS) and deoxyhypusine hydroxylase (DOHH) enzymes. If reduced eIF5A activity is directly linked to polyamine depletion activity, then combination with DHS or DOHH inhibitors (such as ciclopirox) might augment this activity. If protein translation arrest, but not eIF5A modification, correlates with polyamine depletion activity then synergy with eIF5A-independent protein synthesis stressors such as mTorc1 inhibitors (e.g., rapamycin) or homoharringtonine (omecetaxine, FDA-approved for CML) may be anticipated. Similarly, if the contribution of thymidine pool depletion predominates, the combination of DFMO with TS and/or folate metabolism inhibitors could be tested for synergy in pre-clinical models of neuroblastoma. Many such inhibitors are credentialed chemotherapeutics (5-fluorouracil, methotrexate, pemetrexed), and while none are currently used to treat neuroblastoma their anti-tumor activity may be restricted to the setting of provoked polyamine depletion stress.
Defining the key cancer cell-intrinsic mechanisms of activity may also facilitate the development of predictive biomarkers and responder hypotheses aimed at defining the tumor characteristics that predict response to these therapies. These predictions can then be validated in the pre-clinical and clinical setting, facilitating study designs that enrich for patients with a higher probability of deriving benefit. For example, scores of neuroblastoma cell lines have been characterized for genomic alternations, including MYCN and ODC gene copy number. Cell lines with co-amplified ODC1 and MYCN genes, MYCN amplification alone, or no amplification of either gene have been identified and these classes constitute ~8%, 32% and 60% of high-risk tumors, respectively. These can be employed to investigate differential dependencies on cellular polyamines and to identify appropriate biomarkers of polyamine deprivation activity. A biomarker driven pre-clinical program has greater efficiency for combinatorial testing across diverse tumors, and can help prioritize the more resource intensive in vivo drug testing in pre-clinical models that use survival end-points. Although not discussed further, there are other polyamine depletion anti-tumor mechanisms that may be relevant, such as the alteration of global methylation through SAM depletion or selective inhibition of cancer stem-cell functions.
Potential neuroblast extrinsic anti-tumor activities of polyamine deprivation
While substantial data exists that the depletion of polyamines from tumor cells contributes to anti-tumor activity, the contribution of cancer cell-extrinsic activities has recently been highlighted (43). Indeed, findings from neuroblastoma pre-clinical models suggest these extrinsic mechanisms may play a prominent role. In vivo, DFMO dosing regimens resulting in serum concentrations from 150-300 µM leads to robust anti-tumor activity in complementary murine models. Yet these same concentrations only display moderate potency in standard in vitro cytotoxicity and clonogenicity assays against the same cell lines where potent anti-tumor activity is demonstrated in vivo. Further, apparent “pseudo-progression” followed by regression, reminiscent of an immunologic mechanism, is seen both in the TH-MYCN model and in tumors xenografted into T cell defective NK cell-intact nude mice treated with DFMO and celecoxib. In these mice, tumor progression approaching the size necessitating humane culling was followed by complete sustained durable regression in the absence of additional therapy. Pseudo-progression is the term adopted to describe transient tumor growth followed by regression in the setting of effective immunotherapy (53) as these delayed effects may result from the unharnessing of a latent immune response. Given these data we propose it is important that potential effects of polyamine depletion on the solid tumor immunoenvironment be investigated. This is particularly germane as our understanding of the contributions of the tumor microenvironment (TME) to cancer promotion is expanding, and novel therapeutics targeting many of these cancer supportive pathways are under development. As with cancer cell-intrinsic activities, exploring the role of polyamines in this context provides additional opportunities to identify synergistic interactions and potential non-tumor-cell biomarkers, and can inform the extent of polyamine depletion required for these effects.
The development and progression of cancer is promoted by myriad soluble and contact-derived signals obtained from the TME, which consists of tumor cells, tumor-infiltrating leukocytes (TILs), and stromal cells that communicate in a dynamic, bidirectional manner to influence tumor growth, invasion, and metastasis [reviewed in (54)]. These processes depend on the number and function of recruited TILs, which consist of NK cells and effector T cells [such as helper (TH), cytolytic (CTL), and natural killer T (NKT) cells] that mediate anti-tumor immunosurveillance. While neuroblastomas themselves actively engage immune escape pathways through downregulation of HLA class I antigen processing machinery and expression of soluble factors that inhibit immune responses (55), the anti-tumor activity of TILs are also counter-balanced by the collective activities of regulatory T cells (Treg), regulatory B cells (Breg), myeloid-derived suppressor cells (MDSCs) and tumor-associated macrophages (TAMs) (56-58). Once recruited to the tumor additional complexities act to regulate TIL functions, for example, the differentiation of Treg has been shown to be CD8+ T cell dependent (59) through a process that may involve CCL22. In addition to the control exerted by Treg, MDSCs and TAMs can modulate the activity of cytolytic TILs by upregulation of inhibitory ligands (e.g., CTLA4 or PD-L1), downregulation of the expression of MHC and/or co-stimulatory molecules, or production of soluble factors that inhibit effector cell functions.
When compared to lower risk tumors, aggressive neuroblastomas are infiltrated by markedly more inhibitory TAMs polarized to the pro-tumor M2 phenotype (60). Furthermore, a validated poor prognosis signature is enriched for TAM expressed-genes underscoring the functional relevance of this infiltration (61). In addition to TAMs, high-risk neuroblastomas also demonstrate increased inhibitory MDSCs relative to lower risk tumors (62), and the frequency of circulating suppressive Treg are increased (63). In experimental models of neuroblastoma, depletion of these Treg populations results in enhanced anti-tumor immunity (64,65). Collectively, these tumor-promoting cell types may limit the ability of cytotoxic effector (T or NK) cells to control tumor growth, and may induce T cell exhaustion. In an era where the effectiveness of immunotherapeutic approaches to neuroblastoma has been demonstrated, modifying this tumor-permissive TME provides an additional opportunity.
Once recruited to the TME, immune cell activity is further modulated through the actions of soluble mediators (e.g., TGFβ or IL-10) and small molecule metabolites. The latter not only includes polyamines, but also the polyamine precursor amino acid, L-arginine (Figure 2C). Tissue levels of polyamines are determined by the contributions of polyamines from the diet and from the local microbiota, along with polyamines exported from tumor cells as a consequence of enhanced metabolic flux. L-arginine levels in the TME also reflect its abundance in the diet, and the extent of its metabolism towards either polyamines or other nitrogen-containing compounds. Importantly, these two L-arginine-consuming metabolic pathways are in competition and act to cross-repress one another, with the predominant pathway strongly influenced by the phenotype of infiltrating tissue macrophages. Classically activated macrophages (the M1 phenotype, typically activated by IFN-γ) preferentially release inducible NO synthase (iNOS). In turn iNOS upregulation results in release of NO which nitrosylates Odc, a modification that inhibits this rate-limiting enzyme in polyamine synthesis and creates an inflammatory and immunosupportive TME. In contrast, alternatively activated macrophages (M2 phenotype) such as the TAMs that predominate in high-risk neuroblastomas preferentially release arginase 1 to shunt L-arginine metabolism towards polyamine synthesis. Since Odc activity in neuroblastomas is high, the L-ornithine liberated by arginase 1 cleavage of L-arginine is converted by Odc into polyamines. Continued Odc-mediated consumption of L-ornithine prevents it from exerting its negative feedback on arginase 1 activity, while polyamines themselves in turn inhibit iNOS (66). This perpetuates an immunosuppressive TME with inhibition of T cell effectors (CD8+ more so than CD4+ cells) via L-arginine depletion and increased polyamines under the direction of M2 polarized TAMs (44,67).
This depletion of arginine and abundance of polyamines has myriad influences on immune cell activities, many but not all of which are predicted to inhibit anti-tumor immunity. Polyamines, particularly spermine, decrease the ability of monocytes to release pro-inflammatory cytokines (68), thereby modifying the local anti-tumor responsiveness of TILs, and also inhibit IL-2-dependent NK cell activity (69). Consistent with this notion, the cytolytic function of NK cells in a murine carcinoma model were found to be significantly reduced, but this functional deficit was completely restored after therapeutic depletion of polyamines (46). Spermine also induces time and dose-dependent decreases in the adhesion molecule LFA-1 (CD11a and CD18) in peripheral blood lymphocytes, but not monocytes, suggesting that migration of lymphocytes may be selectively altered as well (70). A number of studies suggest that the immune suppressive activity of polyamines to reduce lymphocyte proliferation and IL-2 production, macrophage-mediated tumoricidal activity, neutrophil locomotion, and IL-2-dependent NK cell activity, may all be reversed through the pharmacological depletion of polyamines (45,46,69,71-73). Interestingly, neoadjuvant polyamine depletion prior to tumor resection has at least in one model induced resistance to subsequent tumor re-challenge, suggesting that the effects of this therapy may induce long-lasting effects that could prevent tumor recrudescence (43).
In T cells, L-arginine depletion results in formation of ROS (especially H2O2) and induces decreased functionality (74). This includes downregulation of the CD3ζ chain that is essential for transmittal of activating signals derived from engagement of the T cell receptor, thereby reducing T cell antigen-specific reactivity (67). The CD3ζ chain also associates with CD16, the Fc receptor chain expressed on NK cells, and with the activation receptors NKp30 and NKp46 (75). Since the activation of NK cells depends on the balance of signals imparted via the engagement of surface activating and inhibitory receptors, reductions in expression of the activating receptors may effectively reduce NK-based anti-tumor immunity. Indeed, L-arginine depletion has been shown to reduce NK cell cytotoxicity, cytokine production, and proliferation. Conversely, L-arginine supplementation reduces the expression of certain matrix metalloproteases and increases tumor NO levels, which could, in turn, increase NK cell cytotoxic activity (76).
It is possible that systemic DFMO therapy exerts an anti-tumor effect by depleting polyamines in the TME through the inhibition of Odc activity in both tumor and non-tumor cells, counteracting to some degree the tumor permissive milieu that polyamines have helped to establish (Figure 2D). It is tempting to speculate that the depletion of polyamines in pre-malignant tissues and the preservation of host anti-tumor surveillance serves as the predominant mechanism of chemoprevention of tumors by DFMO. Given the complexity of the immune system in intact animals, and the importance of testing tumors in their autochthonous sites, the TH-MYCN neuroblastoma model provides a more faithful pre-clinical platform for such tests. In a transplantable TH-MYCN model of neuroblastoma (in the C57Bl/6 background) the absence of NK cells but not T cells results in acceleration of tumor growth (77), supporting NK cells as prominent in mediating natural anti-tumor responses in this model. The immune environment of the TH-MYCN model has begun to be characterized with respect to the recruitment and activity of TILs that accompany tumor progression, and these align with those identified in human tumors (78). At inception, tumors are infiltrated with immune effectors of the adaptive immune system like CD8+ T cells but these become proportionately fewer as the tumors progress. In contrast, the proportions of inhibitory M2 TAMs, MDSCs and inhibitory DCs increases 5-fold during tumor progression, leading to a tumor milieu that promotes immune escape. Proof of principle that targeting the tumor immune environment can alter tumor growth kinetics was provided by treating animals with the anti-inflammatory aspirin (acetylsalicylic acid). Low dose aspirin therapy given for ten days around the time of tumor onset and progression led to a significant reduction inhibitory cells of the innate immune system, namely, TAMs, MDSCs and DCs, and reduced tumor volumes at sacrifice (78). It is possible that cyclo-oxygenease inhibitors such as celecoxib or sulindac synergize with DFMO activity through similar interactions on the TME.
Future directions in the development of DFMO and polyamine antagonist regimens for neuroblastoma
The observation that polyamines are required to support cellular proliferation has led to the development of drugs that antagonize polyamine homeostasis for use as anti-tumor agents. While DFMO has shown activity as a single agent against hematolymphoid tumors (79,80) and in combination with chemotherapy for select CNS neoplasms (81), these studies were agnostic to tumor genotype or oncogenic driver. Substantial pre-clinical data now strongly support that DFMO-based therapies may have enhanced activity against tumors with deregulated Myc signaling, such as neuroblastoma. That polyamine depletion therapeutics may target the principal oncogenic driver in neuroblastoma cells while enhancing host anti-tumor immunity by antagonizing the tumor permissive microenvironment makes this therapeutic approach attractive for further development. Current high-risk therapy for neuroblastoma includes induction, consolidation and maintenance phases. The induction phase includes chemotherapy and surgery with the goal of rapidly reducing tumor burden; consolidation therapy includes myeloablative chemoradiotherapy with autologous stem cell support to overcome de novo and acquired therapy resistant tumor cells; and maintenance therapy consists of retinoid-based biotherapy and anti-tumor immunotherapy to target minimal residual disease. In this context, DFMO is currently being developed both as a tumor-targeted agent to synergize with chemotherapy in induction or consolidation therapy, as well as an immune-modulatory agent that may improve host anti-tumor immunity in the maintenance phase, either concurrent with, or following, immunotherapy. Both approaches are biologically plausible and not necessarily mutually exclusive. However, we propose that available pre-clinical models can inform the design of upcoming pivotal Phase 2 and 3 trials by providing a better understanding of the mechanisms of anti-tumor activity for these agents. Further, this approach can facilitate the development of appropriate biomarkers, responder hypotheses, target drug exposures and rational combination therapies that can then be tested in the clinic.
Acknowledgements
Funding: This work is supported by the US Department of Defense W81XWH-10-1-0145 (to M.D.H and S.G.) and the Children’s Neuroblastoma Cancer Foundation (to M.D.H.); The National Health and Medical Research Council (Australia) and Cancer Institute New South Wales (to M.H. and M.D.N).
Footnote
Conflicts of Interest: The authors have no conflicts of interest to declare.
References
- Maris JM, Hogarty MD, Bagatell R, et al. Neuroblastoma. Lancet 2007;369:2106-20. [PubMed]
- Moreno L, Vaidya SJ, Pinkerton CR, et al. Long-term follow-up of children with high-risk neuroblastoma: the ENSG5 trial experience. Pediatr Blood Cancer 2013;60:1135-40. [PubMed]
- Pugh TJ, Morozova O, Attiyeh EF, et al. The genetic landscape of high-risk neuroblastoma. Nat Genet 2013;45:279-84. [PubMed]
- Brodeur GM, Seeger RC, Schwab M, et al. Amplification of N-myc in untreated human neuroblastomas correlates with advanced disease stage. Science 1984;224:1121-4. [PubMed]
- Westermann F, Muth D, Benner A, et al. Distinct transcriptional MYCN/c-MYC activities are associated with spontaneous regression or malignant progression in neuroblastomas. Genome Biology 2008;9:R150. [PubMed]
- Weiss WA, Aldape K, Bishop JM. Targeted expression of NMYC causes neuroblastoma in transgenic mice. The EMBO Journal 1997;16:2985-95. [PubMed]
- Koomoa DL, Yco LP, Borsics T, et al. Ornithine decarboxylase inhibition by alpha-difluoromethylornithine activates opposing signaling pathways via phosphorylation of both Akt/protein kinase B and p27Kip1 in neuroblastoma. Cancer Res 2008;68:9825-31. [PubMed]
- Hogarty MD, Norris MD, Davis K, et al. ODC1 is a critical determinant of MYCN oncogenesis and a therapeutic target in neuroblastoma. Cancer Res 2008;68:9735-45. [PubMed]
- Nilsson JA, Keller UB, Baudino TA, et al. Targeting ornithine decarboxylase in Myc-induced lymphomagenesis prevents tumor formation. Cancer Cell 2005;7:433-44. [PubMed]
- Basuroy UK, Gerner EW. Emerging concepts in targeting the polyamine metabolic pathway in epithelial cancer chemoprevention and chemotherapy. J Biochem 2006;139:27-33. [PubMed]
- Yu AL, Gilman AL, Ozkaynak MF, et al. Anti-GD2 antibody with GM-CSF, interleukin-2, and isotretinoin for neuroblastoma. N Engl J Med 2010;363:1324-34. [PubMed]
- George RE, Sanda T, Hanna M, et al. Activating mutations in ALK provide a therapeutic target in neuroblastoma. Nature 2008;455:975-8. [PubMed]
- Mossé YP, Laudenslager M, Longo L, et al. Identification of ALK as a major familial neuroblastoma predisposition gene. Nature 2008;455:930-5. [PubMed]
- Cheung NK, Zhang J, Lu C, et al. Association of age at diagnosis and genetic mutations in patients with neuroblastoma. JAMA 2012;307:1062-71. [PubMed]
- Sausen M, Leary RJ, Jones S, et al. Integrated genomic analyses identify ARID1A and ARID1B alterations in the childhood cancer neuroblastoma. Nat Genet 2013;45:12-7. [PubMed]
- Seeger RC, Brodeur GM, Sather H, et al. Association of multiple copies of the N-myc oncogene with rapid progression of neuroblastomas. N Engl J Med 1985;313:1111-6. [PubMed]
- Valentijn LJ, Koster J, Haneveld F, et al. Functional MYCN signature predicts outcome of neuroblastoma irrespective of MYCN amplification. Proc Natl Acad Sci U S A 2012;109:19190-5. [PubMed]
- Fredlund E, Ringnér M, Maris JM, et al. High Myc pathway activity and low stage of neuronal differentiation associate with poor outcome in neuroblastoma. Proc Natl Acad Sci U S A 2008;105:14094-9. [PubMed]
- O'Connell BC, Cheung AF, Simkevich CP, et al. A large scale genetic analysis of c-Myc-regulated gene expression patterns. J Biol Chem 2003;278:12563-73. [PubMed]
- Evageliou NF, Hogarty MD. Disrupting polyamine homeostasis as a therapeutic strategy for neuroblastoma. Clin Cancer Res 2009;15:5956-61. [PubMed]
- Andersson G, Heby O. Polyamine and nucleic acid concentrations in Ehrlich ascites carcinoma cells and liver of tumor-bearing mice at various stages of tumor growth. J Natl Cancer Inst 1972;48:165-72. [PubMed]
- Russell D, Snyder SH. Amine synthesis in rapidly growing tissues: ornithine decarboxylase activity in regenerating rat liver, chick embryo, and various tumors. Proc Natl Acad Sci U S A 1968;60:1420-7. [PubMed]
- Casero RA Jr, Marton LJ. Targeting polyamine metabolism and function in cancer and other hyperproliferative diseases. Nat Rev Drug Discov 2007;6:373-90. [PubMed]
- Matsufuji S, Matsufuji T, Miyazaki Y, et al. Autoregulatory frameshifting in decoding mammalian ornithine decarboxylase antizyme. Cell 1995;80:51-60. [PubMed]
- Albeck S, Dym O, Unger T, et al. Crystallographic and biochemical studies revealing the structural basis for antizyme inhibitor function. Protein Sci 2008;17:793-802. [PubMed]
- Justilien V, Walsh MP, Ali SA, et al. The PRKCI and SOX2 oncogenes are coamplified and cooperate to activate Hedgehog signaling in lung squamous cell carcinoma. Cancer Cell 2014;25:139-51. [PubMed]
- Rounbehler RJ, Li W, Hall MA, et al. Targeting ornithine decarboxylase impairs development of MYCN-amplified neuroblastoma. Cancer Res 2009;69:547-53. [PubMed]
- Hansford LM, Thomas WD, Keating JM, et al. Mechanisms of embryonal tumor initiation: distinct roles for MycN expression and MYCN amplification. Proc Natl Acad Sci U S A 2004;101:12664-9. [PubMed]
- Hackett CS, Hodgson JG, Law ME, et al. Genome-wide array CGH analysis of murine neuroblastoma reveals distinct genomic aberrations which parallel those in human tumors. Cancer Res 2003;63:5266-73. [PubMed]
- Moore HC, Wood KM, Jackson MS, et al. Histological profile of tumours from MYCN transgenic mice. J Clin Pathol 2008;61:1098-103. [PubMed]
- Rasmuson A, Segerstrom L, Nethander M, et al. Tumor Development, Growth Characteristics and Spectrum of Genetic Aberrations in the TH-MYCN Mouse Model of Neuroblastoma. PLoS One 2012;7:e51297. [PubMed]
- Bresler SC, Weiser DA, Huwe PJ, et al. ALK mutations confer differential oncogenic activation and sensitivity to ALK inhibition therapy in neuroblastoma. Cancer Cell 2014;26:682-94. [PubMed]
- Keshelava N, Zuo JJ, Chen P, et al. Loss of p53 function confers high-level multidrug resistance in neuroblastoma cell lines. Cancer Res 2001;61:6185-93. [PubMed]
- Ellis LM, Fidler IJ. Finding the tumor copycat: therapy fails, patients don't. Nat Med 2010;16:974-5. [PubMed]
- Meyskens FL Jr, McLaren CE, Pelot D, et al. Difluoromethylornithine plus sulindac for the prevention of sporadic colorectal adenomas: a randomized placebo-controlled, double-blind trial. Cancer Prev Res (Phila) 2008;1:32-8. [PubMed]
- Wallick CJ, Gamper I, Thorne M, et al. Key role for p27Kip1, retinoblastoma protein Rb, and MYCN in polyamine inhibitor-induced G1 cell cycle arrest in MYCN-amplified human neuroblastoma cells. Oncogene 2005;24:5606-18. [PubMed]
- Chen Y, Weeks RS, Burns MR, et al. Combination therapy with 2-difluoromethylornithine and a polyamine transport inhibitor against murine squamous cell carcinoma. Int J Cancer 2006;118:2344-9. [PubMed]
- Boon K, Caron HN, van Asperen R, et al. N-myc enhances the expression of a large set of genes functioning in ribosome biogenesis and protein synthesis. EMBO J 2001;20:1383-93. [PubMed]
- van Riggelen J, Yetil A, Felsher DW. MYC as a regulator of ribosome biogenesis and protein synthesis. Nat Rev Cancer 2010;10:301-9. [PubMed]
- Barna M, Pusic A, Zollo O, et al. Suppression of Myc oncogenic activity by ribosomal protein haploinsufficiency. Nature 2008;456:971-5. [PubMed]
- Pourdehnad M, Truitt ML, Siddiqi IN, et al. Myc and mTOR converge on a common node in protein synthesis control that confers synthetic lethality in Myc-driven cancers. Proc Natl Acad Sci U S A 2013;110:11988-93. [PubMed]
- Wasylishen AR, Chan-Seng-Yue M, Bros C, et al. MYC phosphorylation at novel regulatory regions suppresses transforming activity. Cancer Res 2013;73:6504-15. [PubMed]
- Hayes CS, Shicora AC, Keough MP, et al. Polyamine-blocking therapy reverses immunosuppression in the tumor microenvironment. Cancer Immunol Res 2014;2:274-85. [PubMed]
- Rodriguez PC, Quiceno DG, Zabaleta J, et al. Arginase I production in the tumor microenvironment by mature myeloid cells inhibits T-cell receptor expression and antigen-specific T-cell responses. Cancer Res 2004;64:5839-49. [PubMed]
- Hayes CS, Burns MR, Gilmour SK. Polyamine blockade promotes antitumor immunity. Oncoimmunology 2014;3:e27360. [PubMed]
- Chamaillard L, Quemener V, Havouis R, et al. Polyamine deprivation stimulates natural killer cell activity in cancerous mice. Anticancer Res 1993;13:1027-33. [PubMed]
- Saini P, Eyler DE, Green R, et al. Hypusine-containing protein eIF5A promotes translation elongation. Nature 2009;459:118-21. [PubMed]
- Huang Y, Higginson DS, Hester L, et al. Neuronal growth and survival mediated by eIF5A, a polyamine-modified translation initiation factor. Proc Natl Acad Sci U S A 2007;104:4194-9. [PubMed]
- Landau G, Bercovich Z, Park MH, et al. The role of polyamines in supporting growth of mammalian cells is mediated through their requirement for translation initiation and elongation. J Biol Chem 2010;285:12474-81. [PubMed]
- Mandal S, Mandal A, Johansson HE, et al. Depletion of cellular polyamines, spermidine and spermine, causes a total arrest in translation and growth in mammalian cells. Proc Natl Acad Sci U S A 2013;110:2169-74. [PubMed]
- Witherspoon M, Chen Q, Kopelovich L, et al. Unbiased metabolite profiling indicates that a diminished thymidine pool is the underlying mechanism of colon cancer chemoprevention by alpha-difluoromethylornithine. Cancer Discov 2013;3:1072-81. [PubMed]
- Bistulfi G, Diegelman P, Foster BA, et al. Polyamine biosynthesis impacts cellular folate requirements necessary to maintain S-adenosylmethionine and nucleotide pools. FASEB J 2009;23:2888-97. [PubMed]
- Hales RK, Banchereau J, Ribas A, et al. Assessing oncologic benefit in clinical trials of immunotherapy agents. Ann Oncol 2010;21:1944-51. [PubMed]
- Quail DF, Joyce JA. Microenvironmental regulation of tumor progression and metastasis. Nat Med 2013;19:1423-37. [PubMed]
- Pistoia V, Morandi F, Bianchi G, et al. Immunosuppressive microenvironment in neuroblastoma. Front Oncol 2013;3:167. [PubMed]
- Condeelis J, Pollard JW. Macrophages: obligate partners for tumor cell migration, invasion, and metastasis. Cell 2006;124:263-6. [PubMed]
- Gabrilovich DI, Ostrand-Rosenberg S, Bronte V. Coordinated regulation of myeloid cells by tumours. Nat Rev Immunol 2012;12:253-68. [PubMed]
- Gajewski TF, Schreiber H, Fu YX. Innate and adaptive immune cells in the tumor microenvironment. Nat Immunol 2013;14:1014-22. [PubMed]
- Spranger S, Spaapen RM, Zha Y, et al. Up-regulation of PD-L1, IDO, and T(regs) in the melanoma tumor microenvironment is driven by CD8(+) T cells. Sci Transl Med 2013;5:200ra116.
- Asgharzadeh S, Salo JA, Ji L, et al. Clinical significance of tumor-associated inflammatory cells in metastatic neuroblastoma. J Clin Oncol 2012;30:3525-32. [PubMed]
- Asgharzadeh S, Pique-Regi R, Sposto R, et al. Prognostic significance of gene expression profiles of metastatic neuroblastomas lacking MYCN gene amplification. J Natl Cancer Inst 2006;98:1193-203. [PubMed]
- Bianchi G, Vuerich M, Pellegatti P, et al. ATP/P2X7 axis modulates myeloid-derived suppressor cell functions in neuroblastoma microenvironment. Cell Death Dis 2014;5:e1135. [PubMed]
- Tilak T, Sherawat S, Agarwala S, et al. Circulating T-regulatory cells in neuroblastoma: a pilot prospective study. Pediatr Hematol Oncol 2014;31:717-22. [PubMed]
- Croce M, Corrias MV, Orengo AM, et al. Transient depletion of CD4(+) T cells augments IL-21-based immunotherapy of disseminated neuroblastoma in syngeneic mice. Int J Cancer 2010;127:1141-50. [PubMed]
- Jing W, Yan X, Hallett WH, et al. Depletion of CD25(+) T cells from hematopoietic stem cell grafts increases posttransplantation vaccine-induced immunity to neuroblastoma. Blood 2011;117:6952-62. [PubMed]
- Blachier F, Mignon A, Soubrane O. Polyamines inhibit lipopolysaccharide-induced nitric oxide synthase activity in rat liver cytosol. Nitric Oxide 1997;1:268-72. [PubMed]
- Rodriguez PC, Zea AH, DeSalvo J, et al. L-arginine consumption by macrophages modulates the expression of CD3 zeta chain in T lymphocytes. J Immunol 2003;171:1232-9. [PubMed]
- Zhang B, Bowerman NA, Salama JK, et al. Induced sensitization of tumor stroma leads to eradication of established cancer by T cells. J Exp Med 2007;204:49-55. [PubMed]
- Soda K. The mechanisms by which polyamines accelerate tumor spread. J Exp Clin Cancer Res 2011;30:95. [PubMed]
- Soda K, Kano Y, Nakamura T, et al. Spermine, a natural polyamine, suppresses LFA-1 expression on human lymphocyte. J Immunol 2005;175:237-45. [PubMed]
- Chamaillard L, Catros-Quemener V, Delcros JG, et al. Polyamine deprivation prevents the development of tumour-induced immune suppression. Br J Cancer 1997;76:365-70. [PubMed]
- Chamaillard L, Catros-Quemener V, Moulinoux JP. Synergistic activation of macrophage activity by polyamine deprivation and cyclophosphamide. Anticancer Res 1997;17:1059-65. [PubMed]
- Ferrante A, Maxwell GM, Rencis VO, et al. Inhibition of the respiratory burst of human neutrophils by the polyamine oxidase-polyamine system. Int J Immunopharmacol 1986;8:411-7. [PubMed]
- Kusmartsev S, Nefedova Y, Yoder D, et al. Antigen-specific inhibition of CD8+ T cell response by immature myeloid cells in cancer is mediated by reactive oxygen species. J Immunol 2004;172:989-99. [PubMed]
- Lanier LL. Up on the tightrope: natural killer cell activation and inhibition. Nat Immunol 2008;9:495-502. [PubMed]
- Jyothi MD, Khar A. Induction of nitric oxide production by natural killer cells: its role in tumor cell death. Nitric Oxide 1999;3:409-18. [PubMed]
- Kroesen M, Nierkens S, Ansems M, et al. A transplantable TH-MYCN transgenic tumor model in C57Bl/6 mice for preclinical immunological studies in neuroblastoma. Int J Cancer 2014;134:1335-45. [PubMed]
- Carlson LM, Rasmuson A, Idborg H, et al. Low-dose aspirin delays an inflammatory tumor progression in vivo in a transgenic mouse model of neuroblastoma. Carcinogenesis 2013;34:1081-8. [PubMed]
- Pless M, Belhadj K, Menssen HD, et al. Clinical efficacy, tolerability, and safety of SAM486A, a novel polyamine biosynthesis inhibitor, in patients with relapsed or refractory non-Hodgkin's lymphoma: results from a phase II multicenter study. Clin Cancer Res 2004;10:1299-305. [PubMed]
- Siimes M, Seppanen P, Alhonen-Hongisto L, et al. Synergistic action of two polyamine antimetabolites leads to a rapid therapeutic response in childhood leukemia. Int J Cancer 1981;28:567-70. [PubMed]
- Levin VA, Hess KR, Choucair A, et al. Phase III randomized study of postradiotherapy chemotherapy with combination alpha-difluoromethylornithine-PCV versus PCV for anaplastic gliomas. Clin Cancer Res 2003;9:981-90. [PubMed]