Dynamic variables predict fluid responsiveness in pre-school and school children undergoing neurosurgery: a prospective observational study
Introduction
Judicious intravascular fluid management to achieve optimum cardiac performance is one of the most important hemodynamic goals in pediatric patients, especially in young children undergoing major surgery. Decreased intravascular volume is common in children undergoing neurosurgery because of preoperative fasting, the vasodilatory effects of anesthetics, and significant blood loss. The response rate to blinded volume expansion ranged from 45% to 69% (1). Excess fluid can increase cerebral edema following surgery-related disruption of the blood-brain barrier, and is associated with worse perioperative outcomes (2,3).
Optimally, preload variables should be accurate, continuous, noninvasive, and operator-independent. Dynamic preload variables are those that reflect the cyclical changes in left ventricle stroke volume (SV) induced by positive pressure ventilation. Their values are higher in hypovolemia, when the heart is functioning at the steep portion of the Frank Starling curve. Respiratory variation in aortic blood flow peak velocity (∆Vpeak), pulse pressure variation (PPV), stroke volume variation (SVV), plethysmographic variability index (PVI), dynamic elastance (Eadyn), and respiratory variation in inferior vena cava diameter have been proposed as superior predictors of fluid responsiveness (a surrogate of hypovolemia) in adults compared with static variables in several systematic reviews and meta-analyses (4-8). Children differ from adults in terms of cardiac performance, arterial compliance, chest wall and lung compliance. ∆Vpeak has been confirmed as the only dynamic parameter that consistently predicts the responders to fluid loading in children in various clinical settings (1,9-12). However, the lack of continuity and expert clinicians in echocardiography decreases its utility in routine clinical practice (13).
In contrast to ∆Vpeak, the evidence that continuous dynamic variables predict fluid responsiveness in children is limited with contradicting results (9,14,15). The predictive reliability of SVV extracted from central arterial contour analysis in children has yet to be determined (15). The FloTrac/Vigileo hemodynamic monitoring system (Edwards Lifesciences, Irvine, CA, USA; Software Version 4.02), which is based on arterial pulse contour analysis, refers to an autocalibration algorithm to obtain SV and SVV. Although several pediatric studies examined SVV’s role in predicting fluid responsiveness, no previous studies have evaluated the predictive value of SVV measured using FloTrac/Vigileo in children with mechanical ventilation. PVI is a continuous dynamic variable that reflects periodic variation of the plethysmographic waveform caused by respiration. Clinical investigations into the ability of PVI to predict fluid responsiveness in pediatric populations have demonstrated inconsistent findings (9,15-19). Eadyn is considered as a functional parameter of arterial load (20), which has been less explored with regard to predicting fluid responsiveness in pediatric population (21).
Therefore, the objective of the present study was to evaluate the accuracy and reliability of SVV estimated by the FloTrac/Vigileo system to predict fluid responsiveness after induction in children aged 4–9 years undergoing major neurosurgery. The performances of PVI, PPV, and Eadyn as fluid responsiveness predictors were also assessed. We hypothesized that PVI, SVV, and Eadyn would accurately predict fluid responsiveness, defined by a baseline ∆Vpeak >15% or an increase in mean arterial pressure (MAP) of >10% following fluid loading, while PPV would not. We present the following article in accordance with the STARD reporting checklist (available at https://dx.doi.org/10.21037/tp-21-281).
Methods
This prospective observational study was conducted at Peking University First Hospital between August 2020 and March 2021. The study was conducted in accordance with the Declaration of Helsinki (as revised in 2013). The study was approved by the Ethical Committee of Peking University First Hospital, Beijing, China (Chairperson Prof YY Yu; ethical number: 2020-061). The study was registered prior to patient enrollment with the Chinese Clinical Trial Registry on May 25, 2020 (ChiCTR identifier: ChiCTR2000033245; principal investigator: Lin-Lin Song). And informed consent was taken from all patients’ parent or legal guardian. Children aged 4–9 years scheduled for elective intracranial epileptogenic lesion excision under general anesthesia were approached for assessing the potential eligibility. Patients were excluded if they had congenital heart disease, cardiac arrhythmias, ventricular dysfunction (ejection fraction <50%), underlying pulmonary disease, increased intracranial pressure, or refusal of consent.
Anesthesia management
Anesthesia was induced with propofol 3 mg/kg, continuous infusion of remifentanil 0.2 µg·kg−1·min−1, and sufentanil 0.1 µg/kg as needed. Mechanical ventilation was set in the volume-controlled mode, a vital volume of 8 mL/kg of ideal body weight, an inspiratory:expiratory ratio of 1:2, positive end-expiratory pressure (PEEP) of 4 cmH2O, and respiratory rate titrated to maintain normocapnia during surgery. Following the induction of anesthesia, a peripheral arterial catheter (22–24 G) was inserted in the radial artery, and the arterial line was connected to a FloTrac pressure transducer. The FloTrac transducer was subsequently connected to the Vigileo monitor and a BeneView T9 anesthesia monitor (Mindray Bio-Medical Electronics Corporation, Shenzhen, China), and was zeroed at the mid-axillary level to atmospheric pressure. The Masimo M-LNCS adhesive pulse oximeter sensor was attached to the thumb or index finger of one hand without an intravenous cannula and wrapped with an opaque cover to prevent outside light interference. The sensor was connected to the Masimo Radical-7 oximeter with PVI software (Masimo Corporation, Irvine, California, USA; Software Version 7.8). A forced-air warming system was applied to maintain body temperature >36 °C.
Hemodynamic monitoring
∆Vpeak
The aortic blood flow waveform at the level of the aortic annulus or the left ventricular outflow tract was obtained using pulsed wave Doppler of transthoracic echocardiography (TTE) in the apical five-chamber view. All echocardiography acquisitions were performed by a single investigator, who had previously performed more than 100 TTE measurements. This investigator was blinded to all hemodynamic measurements using other monitoring devices during the experimental protocol. The echo cine-loops recording the aortic blood flow were saved for subsequent offline measurements of ∆Vpeak. ∆Vpeak was calculated as follows:
where Vpeak max and Vpeak min were the maximum and minimum aortic blood flow peak velocities during a single respiratory cycle.
SVV
The FloTrac/Vigileo system comprises a dedicated FloTrac pressure transducer attached to the radial arterial line and the Vigileo monitor connecting the transducer. After the patients’ age, height, weight and sex were entered, SV, cardiac output and SVV were continuously estimated and displayed. The SV was based on that pulse pressure is proportional to the standard deviation of arterial pulse pressure (PPSD). The device calculates SV as
where K is an autocalibration factor that incorporates the quantification of arterial compliance and vascular resistance based on waveform contour analysis and patient characteristics derived from a multivariate regression model. SVV was calculated as follows:
where SVmin and SVmax were the minimum and maximum SVs over a time frame of 20 s.
That means,
K is a constant during SVV calculation within one minute. Therefore,
SVV calculated using FloTrac/Vigileo reflects PPSD’s variation within 20 s. Although K is not validated in pediatric patients, leading to great errors in SV measurements using FloTrac/Vigileo, SVV is dependent on PPSD max and PPSD min within 20 s, which is not affected by inaccurate K. Therefore, SVV values in pediatric patients are as accurate as those in adult patients.
PPV
PPV was automatically calculated and displayed using the anesthesia monitor with the following equation:
where PPmax and PPmin were the maximum and minimum pulse pressures over a time frame of 10 s according to the manufacturer’s algorithm.
PVI
The Masimo Radical-7 oximeter measured pulse oxygen saturation, perfusion index (PI), and PVI noninvasively and continuously based on transcutaneous multiwavelength analysis of plethysmographic waveforms. The PI is the ratio between the infrared pulsatile (alternating current) signal caused by pulsatile arterial flow and the infrared nonpulsatile (direct current) signal, which is affected by skin, nonpulsatile blood, and other tissues. The PVI was calculated as follows:
where PImin and PImax were the minimum and maximum PIs over a time frame of 2–3 min.
Eadyn
Eadyn is considered as a functional parameter of arterial load, which is calculated as PPV/SVV according to previous studies (20).
Experimental protocol and definition of fluid responder
After the induction of anesthesia, fluid loading was started during a hemodynamically stable phase before surgical incision. Fluid loading was conducted with 10 mL/kg of Ringer’s lactate solution over 10 min, and then all variables (except for ∆Vpeak) were measured before and within 5 min following fluid loading prior to surgical incision. ∆Vpeak at baseline >15% identified fluid “responders” (R) and ∆Vpeak ≤15% identified fluid “nonresponders” (NR). We chose a cutoff value of 15% based on the findings of previous studies, which indicated that this difference had clinical significance (10,15,22-24). The other definition of fluid responsiveness examined was an MAP increase of >10% following fluid loading. One anesthesiologist blinded to the echocardiographic measurement managed the status of the patients and the fluid loading protocol over the study period. The ventilator settings and all other therapeutics were unchanged.
Measurements
Offline measurement of ∆Vpeak was performed by the TTE operator and a second echocardiography expert who were blinded to the patient data. The average of the measurements of ∆Vpeak over three consecutive respiratory cycles by two investigators was used for statistical analysis. The following hemodynamic and respiratory parameters were collected: heart rate, systolic arterial pressure (SAP), MAP, SVV, PPV, PI, PVI, end-tidal CO2, peak inspiratory pressure, and plateau pressure. The average of the three consecutive measurements taken at 1-min intervals before and after fluid loading were recorded for statistical analysis.
Sample size calculation
We assumed that the ratio of the numbers between the R and NR groups was 1:2.5 according to the pilot data from our institution. The sample size was determined by assuming that area under the receiver operating characteristic curve (AUROC) of a variable to predict fluid responsiveness was larger than 0.9, compared with the null hypothesis of having an AUROC of 0.5. A minimum of 42 patients with 12 responders and 30 nonresponders were required using a two-tailed t-test with an α-error of 0.05 and power of 0.9. To account for potential dropouts, 46 patients were enrolled.
Statistical analysis
Descriptive statistics are presented as the mean ± standard deviation (SD), median [interquartile range (IQR)], or counts and percentages where appropriate. Variables were compared before and after fluid loading using the paired t-test or Wilcoxon signed-rank test. The differences between responders and nonresponders at baseline and after fluid loading were evaluated using the Student’s t-test or the Mann-Whitney U test. Bonferroni correction was performed for multiple comparisons. Receiver operating characteristic (ROC) curves were generated to assess the ability of hemodynamic variables to identify responders to fluid loading. The best ROC curve threshold was chosen as that which maximized the Youden index. Predictive accuracy was described based on AUROC using the standard terms: poor (0.6–0.7), fair (0.7–0.8), good (0.8–0.9), and excellent (0.9–1.0). The gray zone approach described by Coste et al. (25) was used to determine the range of the predictor within which a conclusive diagnosis regarding fluid responsiveness could not be made with sufficient certainty. Linear regression model was generated to test linear correlations among dynamic variables. Interobserver reproducibility for ∆Vpeak was assessed using the intraclass correlation coefficient (ICC). The least significant change (LSC) was calculated from the coefficient of variation to characterize the minimum ∆Vpeak change to detect a real change. The performance of predictors in predicting fluid responsiveness with an alternative ∆Vpeak threshold other than 15% was also explored. A sensitivity analysis of the accuracy of the dynamic preload variables was conducted in patients aged between 4 and 6 years. P<0.05 was considered statistically significant. All statistical analyzes were performed using SPSS software (SPSS Version 26, Chicago, IL, USA) and MedCalc software (MedCalc Version 19.8, Ostend, Belgium).
Results
During the study period, 46 patients were eligible for the enrollment (Figure 1). Two patients were excluded because of unavailable Vigileo monitors or protocol violation. Therefore, 44 patients were included in the final analysis. The clinical characteristics of the cohort are shown in Table 1. No patients received vasoactive medication during the period of the experimental protocol.
Table 1
Characteristic | Overall, n=44 | R, n=14 | NR, n=30 | P |
---|---|---|---|---|
Age, mo | 72 [60–96] | 72 [60–108] | 72 [60–96] | 0.824 |
Female, n (%) | 18 [40.9] | 6 [46.2] | 12 [38.7] | 0.737 |
Height, cm | 122 [112–130] | 121 [110–130] | 125 [112–128] | 0.847 |
Weight, kg | 25 [21–27] | 25 [21–30] | 24 [21–26] | 0.469 |
BMI, kg/m2 | 16.7 [15.4–17.7] | 17.5 [16.1–18.7] | 16.4 [15.4–17.7] | 0.142 |
Ventilatory frequency, cycles/min | 16 [15–18] | 16 [15–18] | 16 [15–18] | 0.761 |
Peak inspiratory pressure, cmH2O | 14 [12–15] | 14 [13–15] | 14 [12–15] | 0.774 |
Plateau pressure, cmH2O | 8 [7–9] | 8 [8–9] | 8 [7–9] | 0.947 |
End-tidal CO2 partial pressure | 35 [32–36] | 35 [32–36] | 35 [33–36] | 0.672 |
End-tidal sevoflurane concentration | 0.8 [0.6–1.0] | 1.0 [0.5–1.1] | 0.8 [0.7–1.0] | 0.875 |
Infusion rate of propofol, mg·kg−1·h−1 | 6.0 [5.0–7.0] | 6.0 [5.0–7.0] | 6.0 [5.0–7.0] | 0.913 |
Infusion rate of remifentanil, μg·kg−1·min−1 | 0.13 [0.11–0.15] | 0.13 [0.11–0.15] | 0.14 [0.12–0.15] | 0.221 |
Nasopharyngeal Temperature, °C | 36.2 [36.1–36.5] | 36.2 [36.1–36.4] | 36.2 [36.1–36.5] | 0.937 |
HR/ventilatory frequency | 4.4 [4.1–4.8] | 4.3 [4.1–4.8] | 4.4 [3.7–4.8] | 0.867 |
*P<0.05, R vs. NR. R, responder; NR, nonresponder; BMI, body mass index; HR, heart rate.
Of the patients included, 14 (31.8%) showed a ∆Vpeak >15% before fluid loading and were defined as responders. The cohort’s median (IQR) ∆Vpeak at baseline was 9.7% (6.5% to 15.8%). R had a higher baseline ∆Vpeak than NR [18.2% (16.0% to 22.2%) vs. 7.9% (6.3% to 10.3%); P<0.001]. Demographic data and clinical characteristics revealed no differences between R and NR (Table 1).
Twenty (45.5%) showed an increase in MAP of >10% following fluid loading and were defined as responders. The median (IQR) increase in MAP was 9.3% (0.6–18.5%). R had a greater increase in MAP than NR [15.2% (10.2–18.5%) vs. 6.3% (−2.7–12.9%); P<0.001].
Hemodynamics during fluid loading
Before fluid loading, only the PVI value was significantly different between R and NR (P=0.017) (Table 2). Fluid loading significantly changed MAP, PVI, PPV, and SVV in both R and NR. However, SAP increased following fluid loading in R only. The R group showed a significantly greater absolute change in PPV and SVV after fluid loading from baseline compared with the NR group {absolute PPV change: 3 [1–4] and 4 [3–5] for NR and R, respectively, P=0.021; SVV: absolute SVV change: 2 [1–4] and 3 [3–5] for NR and R, respectively, P=0.040} (Figure 2). There was strong correlation between PVI and ∆Vpeak at baseline (r=0.37, P=0.015) (Figure 3).
Table 2
Variable | R | NR | P R vs. NR, before | P R vs. NR, after | |||||
---|---|---|---|---|---|---|---|---|---|
Before fluid loading | After fluid loading | P before vs. after | Before fluid loading | After fluid loading | P before vs. after | ||||
HR, beats/min | 73 [70–74] | 62 [56–76] | 0.470 | 73 [62–86] | 61 [53–83] | 0.062 | 0.754 | 0.941 | |
SAP, mmHg | 100 [93–107] | 107 [98–121] | 0.003* | 98 [91–120] | 104 [99–120] | 0.043 | 0.743 | 0.412 | |
MAP, mmHg | 69 [64–74] | 76 [71–84] | 0.002* | 68 [65–81] | 74 [66–86] | 0.008* | 0.732 | 0.549 | |
PPV, % | 10 [9–11] | 7 [5–8] | <0.001* | 9 [8–11] | 7 [7–8] | <0.001* | 0.451 | 0.262 | |
SVV, % | 9 [7–14] | 6 [4–7] | <0.001* | 8 [6–10] | 6 [4–8] | <0.001* | 0.483 | 0.651 | |
PVI, % | 16 [14–25] | 13 [10–17] | 0.008* | 12 [12–18] | 10 [8–16] | 0.001* | 0.017** | 0.373 | |
PI, % | 3.7 [3.3–5.9] | 5.0 [3.5–7.1] | 0.090 | 3.8 [3.2–6.9] | 5.4 [3.6–7.0] | 0.057 | 0.797 | 0.599 | |
Eadyn | 1.1 [1.0–1.3] | 1.2 [1.0–1.4] | 0.197 | 1.2 [1.1–1.3] | 1.3 [1.1–1.5] | 0.136 | 0.391 | 0.588 |
*P<0.025, before vs. after fluid loading. **P<0.025, R vs. NR. Bonferroni correction for multiple comparisons (twice per variable) decreased the threshold for significance to 0.025. R, responder; NR, nonresponder; Eadyn, dynamic arterial elastance; HR, heart rate; SAP, systolic arterial pressure; MAP, mean arterial pressure; PPV, pulse pressure variation; SVV, stroke volume variation; PVI, plethysmographic variation index; PI, perfusion index.
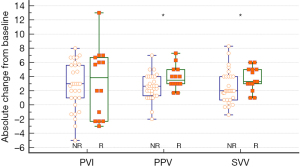
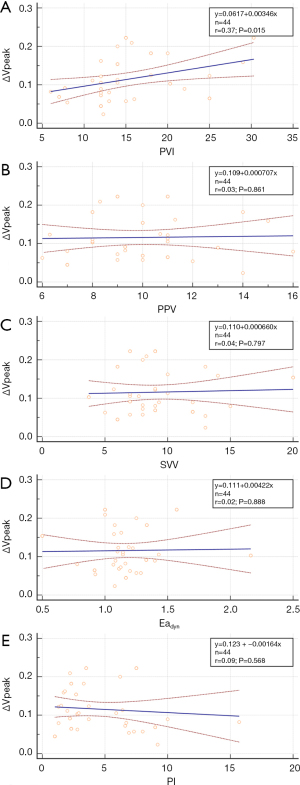
Prediction of fluid responsiveness defined by baseline ∆Vpeak
The results of ROC curve analysis for hemodynamic variables to discriminate between R and NR with fluid responsiveness definition of a baseline ∆Vpeak >15% are summarized in Table 3 and Figure 4A. Baseline PVI showed fair diagnostic accuracy for fluid responsiveness defined by a baseline ∆Vpeak >15%, with an AUROC value of 0.735 (95% CI: 0.560–0.857, P=0.002). A baseline PVI cutoff value of >13% (95% CI: 12–18%) predicted fluid responsiveness with a sensitivity of 93% and a specificity of 60%. The gray zone of PVI ranged between 12% and 20%, which included 43.8% of patients. Baseline PPV, SVV, PI, and Eadyn were not capable of predicting fluid responsiveness.
Table 3
AUROC (95% CI) | P | Sensitivity (%) | Specificity (%) | Optimal threshold | |
---|---|---|---|---|---|
Overall (n=44, R=14, NR=30) | |||||
PVI | 0.735 (0.560–0.857) | 0.002* | 93 | 60 | 13% |
PPV | 0.560 (0.395–0.738) | 0.509 | 100 | 20 | 7% |
SVV | 0.608 (0.425–0.785) | 0.245 | 29 | 90 | 12% |
Eadyn | 0.581 (0.347–0.770) | 0.447 | 57 | 77 | 1.1 |
PI | 0.514 (0.345–0.678) | 0.872 | 0 | 80 | 1.5% |
4–6 age subgroup (n=28, R=10, NR=18) | |||||
PVI | 0.792 (0.580–0.925) | <0.001* | 90 | 72 | 13% |
PPV | 0.533 (0.272–0.746) | 0.785 | 100 | 22 | 7% |
SVV | 0.514 (0.275–0.708) | 0.905 | 90 | 22 | 10% |
Eadyn | 0.561 (0.289–0.816) | 0.650 | 40 | 100 | 1.3 |
PI | 0.644 (0.426–0.831) | 0.169 | 100 | 33 | 1.5% |
*P<0.05, compared with an AUROC of 0.5. AUROC, area under the receiver operating characteristic curve; CI, confidence interval; R, responder; NR, nonresponder; Eadyn, dynamic arterial elastance; PPV, pulse pressure variation; SVV, stroke volume variation; PI, perfusion index; PVI, plethysmographic variation index; ∆Vpeak, respiratory variation in aortic blood flow peak velocity.
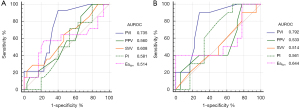
Sensitivity analysis
Ten (35.7%) of 28 patients in the 4–6 age subgroup showed fluid responsiveness defined by a baseline ∆Vpeak >15%, compared with 4 (25.0%) of 16 patients in the 7–9 age subgroup (P=0.463). In the 4–6 subgroup analysis, the results were essentially comparable with those of the whole cohort (Table 3 and Figure 4B). PVI was a good predictor of fluid responsiveness with an improved AUROC of 0.792 in the patients aged 4–6 years and no change was detected in terms of the optimal PVI threshold value. PPV, SVV, PI, and Eadyn were not predictors of fluid responsiveness in the 4–6 age group.
The diagnostic accuracy and the cutoff threshold of PVI according to incremental ∆Vpeak cutoff values to define a positive fluid response did not differ significantly (Table 4).
Table 4
∆Vpeak thresholds (%) | R/NR | AUROC (95% CI) | P | Sensitivity (%) | Specificity (%) | Optimal threshold (%) |
---|---|---|---|---|---|---|
10 | 23/21 | 0.735 (0.547–0.860) | 0.003* | 83 | 71 | 13 |
11 | 19/25 | 0.707 (0.523–0.844) | 0.009* | 84 | 64 | 13 |
12 | 17/27 | 0.749 (0.566–0.873) | <0.001* | 88 | 63 | 13 |
13–15 | 14/30 | 0.735 (0.560–0.857) | 0.002* | 93 | 60 | 13 |
16 | 11/33 | 0.720 (0.558–0.862) | 0.006* | 82 | 67 | 14 |
17–18 | 10/34 | 0.766 (0.598–0.886) | <0.001* | 90 | 68 | 14 |
19 | 7/37 | 0.732 (0.569–0.880) | 0.010* | 100 | 51 | 13 |
20 | 6/38 | 0.765 (0.570–0.921) | 0.003* | 100 | 63 | 14 |
*P<0.05, compared with an AUROC of 0.5. R, responder; NR, nonresponder; ROC, receiver operating characteristic; AUROC, area under the receiver operating characteristic curve; CI, confidence interval; PVI, plethysmographic variability index; ∆Vpeak, respiratory variation in aortic blood flow peak velocity.
Prediction of fluid responsiveness defined by a MAP increase
Baseline ∆Vpeak and SVV demonstrated fair predictive values for fluid responsiveness defined by an increase in MAP of >10% following fluid loading, with the optimal cutoff baseline ∆Vpeak of 12.5% and SVV of 9 (AUROC =0.758 and 0.715, respectively) (Table 5 and Figure 5). PPV, PVI, and Eadyn were poor predictors for a MAP increase of >10% following fluid loading.
Table 5
AUROC (95% CI) | P | Sensitivity (%) | Specificity (%) | Optimal threshold | |
---|---|---|---|---|---|
∆Vpeak | 0.758 (0.588–0.891) | <0.001* | 60 | 92 | 12.5% |
PVI | 0.667 (0.475–0.814) | 0.045* | 80 | 63 | 13% |
PPV | 0.686 (0.511–0.839) | 0.023* | 55 | 79 | 11% |
SVV | 0.715 (0.533–0.849) | 0.006* | 50 | 88 | 9% |
PI | 0.518 (0.324–0.689) | 0.844 | 85 | 38 | 2.2% |
Eadyn | 0.686 (0.501–0.859) | 0.044* | 55 | 96 | 1.1 |
*P<0.05, compared with an AUROC of 0.5. AUROC, area under the receiver operating characteristic curve; CI, confidence interval; R, responder; NR, nonresponder; Eadyn, dynamic arterial elastance; PPV, pulse pressure variation; SVV, stroke volume variation; PI, perfusion index; PVI, plethysmographic variation index; ∆Vpeak, respiratory variation in aortic blood flow peak velocity.
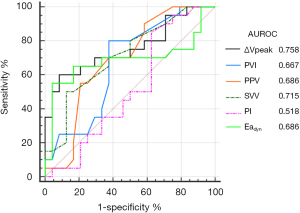
Agreement analysis
Interobserver reproducibility for the measurements of baseline aortic blood flow peak velocity were acceptable (ICC 0.95, 95% CI: 0.94–0.96). The coefficient of variations for baseline ∆Vpeak from two observers were 10.0% (95% CI: 7.5–12.5%) and 8.7% (95% CI: 6.3–11.1%), respectively. The mean LSC for baseline aortic blood flow peak velocity was 13.2%.
Discussion and conclusion
Our results demonstrated that, baseline PVI was a fair dynamic predictor of fluid responsiveness after induction defined by a baseline ∆Vpeak of >15%, in children aged 4–9 years undergoing epileptogenic lesion excision. Baseline ∆Vpeak and SVV had fair predictive values for a response of a MAP increase of >10% following fluid loading.
As a dynamic variable based on volume, not pressure, conflicting results have been obtained among investigations on the predictive values of PVI in the pediatric population. Two positive studies in children aged <10 years receiving neurosurgery or open surgery revealed that, PVI was accurate when used to predict fluid responsiveness (9,16). They were conducted in otherwise healthy patients similar to our cohort, and the results also concurred with ours. In contrast, a previous study investigated PVI’s ability to predict a positive response to a bolus of 20 mL/kg normal saline after induction in neurosurgical patients aged 0–6 and 6–14 years, and failed to demonstrate its predictive value (15). The discrepancies observed between the above study and our study can be explained by the heterogeneity of the studied population and different interventions. In addition, their study may be underpowered by insufficient subject numbers in each age strata. Volume-based PVI is expected to be more sensitive to ventilator-induced changes than pressure-derived PPV or SVV in children. In addition to periodic change in SV during mechanical ventilation, the increased resistance to venous return during inspiration may increase the volume of venous blood, thus exaggerating the decrease in plethysmographic wave amplitude (26). However, it should be noted that PVI is affected by many factors related to PI, such as vasoactive drugs, hypothermia, anxiety, cardiac arrhythmia, and sampling site, contacting force. A PI >4% improves the validity of PVI values (27). Fifty-three percent of our cohort showed PI values <4%, and the predictive reliability of PVI in the present study must be interpreted with caution. In addition, its gray zone includes a considerable number of patients.
Hemodynamic monitoring techniques measuring cardiac out and SVV currently assessed in pediatric population include esophageal Doppler technique (USCOM), transesophageal echocardiography, thoracic electrical bioimpedance devices, pulse contour analysis (PiCCO and Mostcare), and bioreactance devices (NICOM), with varying diagnostic values of SVV in predicting fluid responsiveness. Because of the diverse SVV algorithms in proprietary monitors, interventions, and patient characteristics, the pediatric studies investigating the predictive value of the SVV for fluid responsiveness are almost incomparable to each other (14,22,28-31). A meta-analysis of SVV application in children examined studies using PiCCO, USCOM, NICOM, and Mostcare, and concluded that, SVV was of diagnostic value in predicting fluid responsiveness in children under mechanical ventilation (32). However, authors considered that further studies are needed to confirm the predictive values of SVV because of high heterogeneity of studies and mainly cardiosurgical and intensive care pediatric patients included. One positive study measuring SVV using NICOM enrolled patients similar to the present study by including patients aged 0–16 years undergoing craniosynostosis repair (29). The discrepancies observed between the above study and ours can be explained by age difference in the studied populations, type and volume of fluid infused, and proprietary monitors. We applied a tidal volume of 8 mL/kg with a PEEP of 4 cmH2O as clinical routines to induce a minimum meaningful intrathoracic pressure swing. It might be possible that higher values of a tidal volume and no PEEP applied would improve the predictive performance of SVV regarding fluid responsiveness. Increased arterial compliance in children, compared with adults, partially absorbs the magnitude of change in arterial blood pressure caused by an increase in SV during positive pressure ventilation. That may partly explain PPV and SVV’s failure to predict fluid responsiveness defined by a baseline ∆Vpeak of >15%.
Although baseline PPV was not found to be useful for predicting fluid responsiveness after anesthesia induction in our study, responders had great absolute changes in the PPV and SVV values from baseline compared with nonresponders. The mini-fluid challenge is a clinical method of predicting fluid responsiveness by rapidly infusing small amount of intravenous fluids, and assessing its systemically hemodynamic effect. This method is meant to predict if a patient will respond to a subsequent, larger fluid challenge with a significant increase in SV. Further investigation is required to evaluate, whether a mini-fluid challenge test determining the change in PPV before and after fluid challenge would be helpful, in discriminating fluid responsiveness in children with a PVI value in gray zone and guiding optimal perioperative fluid management in children (33).
The present study demonstrated that the patients aged 4–6 years had an improved AUROC of PVI for predicting fluid responsiveness compared to that in the overall patients, although this result might be underpowered by limited patient numbers. The pediatric population includes different age groups that may vary in dynamic variables’ predictive reliabilities and optimal cutoffs regarding fluid responsiveness. This is partly because of the children’s varying physiologic characteristics with increasing age, related to cardiopulmonary development and vascular compliance change (34-36). More subjects are required to establish age-specific predictors and optimal cutoff values.
The adequate intraoperative MAP is crucial for modern anesthetic care (37). Arterial pressure is dependent on the interaction between left ventricular SV and arterial tone. Given comparative arterial tones (reflected by Eadyn) between R and NR, arterial pressure might be significantly associated with stroke volume in the present study. That may explain why baseline ∆Vpeak was found to be a fair predictor of a MAP increase following fluid loading in our cohort. We also found that baseline SVV, not PVI, fairly predicted a MAP increase following fluid challenge. Different from volume-based PVI, SVV calculated using FloTrac/Vigileo is pressure-derived, which reflects the variation of standard deviation in pulse pressure. Further studies are needed to explore the relationship between ∆Vpeak or SVV and MAP in pediatric population.
The most common reference standard for the definition of fluid responsiveness is a change in SV of greater than 15% as measured by transesophageal or transthoracic echocardiography. ∆Vpeak is the only variable to consistently predict fluid responsiveness in children with an AUROC of 0.8–1.0 (1,9-12). We decided to use baseline ∆Vpeak to distinguish responders from nonresponders, instead of SV measurement. One single echocardiographic measurement of ∆Vpeak has the potential to reduce intra- and interobserver variability and echocardiographic measurement errors related to two operator-dependent measurements required for SV determination. No unequivocal cutoff values for baseline ∆Vpeak to define fluid responsiveness have been determined in the literature to date. The reported threshold values vary considerably across the studies from 7% to 20% (1,9-12). Two previous studies demonstrated that ∆Vpeak threshold values of 10% and 11% respectively reliably predicted an increase in SV of >15% to fluid loading in a comparable patient population (9,15). In the present study, we arbitrarily defined responders with baseline ∆Vpeak >15%, which is a high limit of the cutoff values previously reported, aiming to avoid falsely positive fluid responsiveness detection. In addition, 15% is more than the expected error of echocardiographic ∆Vpeak measurements and is generally considered clinically relevant. A baseline ∆Vpeak threshold value of 15% was reasonably justified by the LSC of 13.2%.
Limitations
First, the current study had a small cohort and was conducted in a single tertiary hospital. Second, although decreased intravascular volume after anesthesia induction is common, the majority of elective patients are no more than moderately dehydrated with no or mild clinical signs of volume depletion. Pediatric patients with significant clinical signs of hypovolemia might yield different results. Last, the FloTrac/Vigileo cardiac output monitoring system is not validated for cardiac index monitoring in the pediatric setting. However, according to the SV formula (SV = PPSD × K), the imprecise SV measurements come from an inaccurate K. Any error in this method would only affect the precision of the maximum and minimum values of SV, but not the ratio of (SVmax–SVmin)/SVmean. SVV is therefore not subject to algorithm errors.
In conclusion, baseline PVI showed fair reliability to predict fluid responsiveness, defined by a baseline ∆Vpeak of >15%, after anesthesia induction in mechanically ventilated children aged 4–9 years undergoing major neurosurgery. Baseline PPV, FloTrac/Vigileo-derived SVV, and Eadyn did not accurately predict fluid responsiveness reflected by a baseline ∆Vpeak of >15%. Baseline ∆Vpeak and SVV were fair predictors for an increase in MAP following fluid loading.
Acknowledgments
Funding: None.
Footnote
Reporting Checklist: The authors have completed the STARD reporting checklist. Available at https://dx.doi.org/10.21037/tp-21-281
Data Sharing Statement: available at https://dx.doi.org/10.21037/tp-21-281
Peer Review File: Available at https://dx.doi.org/10.21037/tp-21-281
Conflicts of Interest: All authors have completed the ICMJE uniform disclosure form (available at https://dx.doi.org/10.21037/tp-21-281). The authors have no conflicts of interest to declare.
Ethical Statement: The authors are accountable for all aspects of the work in ensuring that questions related to the accuracy or integrity of any part of the work are appropriately investigated and resolved. The study was conducted in accordance with the Declaration of Helsinki (as revised in 2013). The study was approved by the Ethical Committee of Peking University First Hospital, Beijing, China (Chairperson Prof YY Yu; ethical number: 2020-061). The study was registered prior to patient enrollment with the Chinese Clinical Trial Registry on May 25, 2020 (ChiCTR identifier: ChiCTR2000033245; principal investigator: Lin-Lin Song). And informed consent was taken from all patients’ parent or legal guardian.
Open Access Statement: This is an Open Access article distributed in accordance with the Creative Commons Attribution-NonCommercial-NoDerivs 4.0 International License (CC BY-NC-ND 4.0), which permits the non-commercial replication and distribution of the article with the strict proviso that no changes or edits are made and the original work is properly cited (including links to both the formal publication through the relevant DOI and the license). See: https://creativecommons.org/licenses/by-nc-nd/4.0/.
References
- Desgranges FP, Desebbe O, Pereira de Souza Neto E, et al. Respiratory variation in aortic blood flow peak velocity to predict fluid responsiveness in mechanically ventilated children: a systematic review and meta-analysis. Paediatr Anaesth 2016;26:37-47. [Crossref] [PubMed]
- Xia J, He Z, Cao X, et al. The brain relaxation and cerebral metabolism in stroke volume variation-directed fluid therapy during supratentorial tumors resection: crystalloid solution versus colloid solution. J Neurosurg Anesthesiol 2014;26:320-7. [Crossref] [PubMed]
- Luo J, Xue J, Liu J, et al. Goal-directed fluid restriction during brain surgery: a prospective randomized controlled trial. Ann Intensive Care 2017;7:16. [Crossref] [PubMed]
- Loupec T, Nanadoumgar H, Frasca D, et al. Pleth variability index predicts fluid responsiveness in critically ill patients. Crit Care Med 2011;39:294-9. [Crossref] [PubMed]
- Wiesenack C, Fiegl C, Keyser A, et al. Assessment of fluid responsiveness in mechanically ventilated cardiac surgical patients. Eur J Anaesthesiol 2005;22:658-65. [Crossref] [PubMed]
- Rex S, Brose S, Metzelder S, et al. Prediction of fluid responsiveness in patients during cardiac surgery. Br J Anaesth 2004;93:782-8. [Crossref] [PubMed]
- Sandroni C, Cavallaro F, Marano C, et al. Accuracy of plethysmographic indices as predictors of fluid responsiveness in mechanically ventilated adults: a systematic review and meta-analysis. Intensive Care Med 2012;38:1429-37. [Crossref] [PubMed]
- Marik PE, Cavallazzi R, Vasu T, et al. Dynamic changes in arterial waveform derived variables and fluid responsiveness in mechanically ventilated patients: a systematic review of the literature. Crit Care Med 2009;37:2642-7. [Crossref] [PubMed]
- Byon HJ, Lim CW, Lee JH, et al. Prediction of fluid responsiveness in mechanically ventilated children undergoing neurosurgery. Br J Anaesth 2013;110:586-91. [Crossref] [PubMed]
- Choi DY, Kwak HJ, Park HY, et al. Respiratory variation in aortic blood flow velocity as a predictor of fluid responsiveness in children after repair of ventricular septal defect. Pediatr Cardiol 2010;31:1166-70. [Crossref] [PubMed]
- Durand P, Chevret L, Essouri S, et al. Respiratory variations in aortic blood flow predict fluid responsiveness in ventilated children. Intensive Care Med 2008;34:888-94. [Crossref] [PubMed]
- Lee JY, Kim JY, Choi CH, et al. The ability of stroke volume variation measured by a noninvasive cardiac output monitor to predict fluid responsiveness in mechanically ventilated children. Pediatr Cardiol 2014;35:289-94. [Crossref] [PubMed]
- Vignon P, Repessé X, Bégot E, et al. Comparison of Echocardiographic Indices Used to Predict Fluid Responsiveness in Ventilated Patients. Am J Respir Crit Care Med 2017;195:1022-32. [Crossref] [PubMed]
- Renner J, Broch O, Duetschke P, et al. Prediction of fluid responsiveness in infants and neonates undergoing congenital heart surgery. Br J Anaesth 2012;108:108-15. [Crossref] [PubMed]
- Pereira de Souza Neto E, Grousson S, Duflo F, et al. Predicting fluid responsiveness in mechanically ventilated children under general anaesthesia using dynamic parameters and transthoracic echocardiography. Br J Anaesth 2011;106:856-64. [Crossref] [PubMed]
- Julien F, Hilly J, Sallah TB, et al. Plethysmographic variability index (PVI) accuracy in predicting fluid responsiveness in anesthetized children. Paediatr Anaesth 2013;23:536-46. [Crossref] [PubMed]
- Chen PH, Chan KC, Liao MH, et al. Accuracy of dynamic preload variables for predicting fluid responsiveness in patients with pediatric liver cirrhosis: A prospective study. Paediatr Anaesth 2020;30:455-61. [Crossref] [PubMed]
- Choi SN, Ji SH, Jang YE, et al. Predicting hypotension during anesthesia: Variation in pulse oximetry plethysmography predicts propofol-induced hypotension in children. Paediatr Anaesth 2021;31:894-901. [Crossref] [PubMed]
- Kim EH, Lee JH, Jang YE, et al. Prediction of fluid responsiveness using lung recruitment manoeuvre in paediatric patients receiving lung-protective ventilation: A prospective observational study. Eur J Anaesthesiol 2021;38:452-8. [Crossref] [PubMed]
- Zhang F, He ST, Zhang Y, et al. Comparison of Two Malnutrition Assessment Scales in Predicting Postoperative Complications in Elderly Patients Undergoing Noncardiac Surgery. Front Public Health 2021;9:694368 [Crossref] [PubMed]
- Lee JH, Kwon YL, Na JH, et al. Is dynamic arterial elastance a predictor of an increase in blood pressure after fluid administration in pediatric patients with hypotension? Reanalysis of prospective observational studies. Paediatr Anaesth 2020;30:34-42. [Crossref] [PubMed]
- Lee JH, No HJ, Song IK, et al. Prediction of fluid responsiveness using a non-invasive cardiac output monitor in children undergoing cardiac surgery. Br J Anaesth 2015;115:38-44. [Crossref] [PubMed]
- Morparia KG, Reddy SK, Olivieri LJ, et al. Respiratory variation in peak aortic velocity accurately predicts fluid responsiveness in children undergoing neurosurgery under general anesthesia. J Clin Monit Comput 2018;32:221-6. [Crossref] [PubMed]
- Renner J, Broch O, Gruenewald M, et al. Non-invasive prediction of fluid responsiveness in infants using pleth variability index. Anaesthesia 2011;66:582-9. [Crossref] [PubMed]
- Coste J, Pouchot J. A grey zone for quantitative diagnostic and screening tests. Int J Epidemiol 2003;32:304-13. [Crossref] [PubMed]
- Dorlas JC, Nijboer JA. Photo-electric plethysmography as a monitoring device in anaesthesia. Application and interpretation. Br J Anaesth 1985;57:524-30. [Crossref] [PubMed]
- Broch O, Bein B, Gruenewald M, et al. Accuracy of the pleth variability index to predict fluid responsiveness depends on the perfusion index. Acta Anaesthesiol Scand 2011;55:686-93. [Crossref] [PubMed]
- Saxena R, Durward A, Steeley S, et al. Predicting fluid responsiveness in 100 critically ill children: the effect of baseline contractility. Intensive Care Med 2015;41:2161-9. [Crossref] [PubMed]
- Vergnaud E, Vidal C, Verchère J, et al. Stroke volume variation and indexed stroke volume measured using bioreactance predict fluid responsiveness in postoperative children. Br J Anaesth 2015;114:103-9. [Crossref] [PubMed]
- McLean JR, Inwald DP. The utility of stroke volume variability as a predictor of fluid responsiveness in critically ill children: a pilot study. Intensive Care Med 2014;40:288-9. [Crossref] [PubMed]
- Song Y, Hou H, Bai J, et al. Prediction of Fluid Responsiveness by Stroke Volume Variation in Children Undergoing Fontan Operation. Biomed Res Int 2020;2020:2595960 [Crossref] [PubMed]
- Yi L, Liu Z, Qiao L, et al. Does stroke volume variation predict fluid responsiveness in children: A systematic review and meta-analysis. PLoS One 2017;12:e0177590 [Crossref] [PubMed]
- Zorio V, Lebreton T, Desgranges FP, et al. Does a two-minute mini-fluid challenge predict fluid responsiveness in pediatric patients under general anesthesia? Paediatr Anaesth 2020;30:161-7. [Crossref] [PubMed]
- ROACH MR. BURTON AC. The effect of age on the elasticity of human iliac arteries. Can J Biochem Physiol 1959;37:557-70. [Crossref] [PubMed]
- Senzaki H, Akagi M, Hishi T, et al. Age-associated changes in arterial elastic properties in children. Eur J Pediatr 2002;161:547-51. [Crossref] [PubMed]
- Papastamelos C, Panitch HB, England SE, et al. Developmental changes in chest wall compliance in infancy and early childhood. J Appl Physiol (1985) 1995;78:179-84. [PubMed]
- Sessler DI, Bloomstone JA, Aronson S, et al. Perioperative Quality Initiative consensus statement on intraoperative blood pressure, risk and outcomes for elective surgery. Br J Anaesth 2019;122:563-74. [Crossref] [PubMed]