The protective effect of MiR-27a on the neonatal hypoxic-ischemic encephalopathy by targeting FOXO1 in rats
Introduction
Neonatal hypoxic-ischemic encephalopathy (HIE), a kind of hypoxic-ischemic brain damage caused by perinatal asphyxia, is the most crucial cause of neonatal death and long-term neurological dysfunction in children (1-3). Many studies have been carried on the occurrence, progress, regulation, and other mechanisms of hypoxic-ischemic brain damage (4,5). The main pathophysiological changes are necrosis of nerve cells in the acute phase and apoptosis of nerve cells after reperfusion injury, mainly involving mitochondrial injury and endoplasmic reticulum (ER) stress, production of free radicals, inflammation, and immune response (6-9). Although researchers are continuing to explore the treatment strategy for HIE, it has been found that 40–50% of children who receive treatment for HIE die or experience adverse sequelae of the nervous system, such as cerebral palsy, visual and auditory impairment, epilepsy, developmental retardation, autism, and so on (10-12). The prognosis of severe HIE is worse than that of more mild cases (13). Therefore, the exploration of the pathogenesis of neonatal HIE and the discovery of new treatment options have been a hot research topic worldwide (14).
MicroRNA (miRNA), a research hotspot in recent years, is a kind of endogenous small non coding RNA, which is generally composed of 18–24 nucleotides (15). It can silence the expression of a target gene by binding to the 3' untranslated region (3’UTR) of the target gene sequence to inhibit its transcriptional activity (16). In the human genome, there are about 700 miRNAs, which are mainly involved in the regulation of cell differentiation, metabolism, apoptosis, angiogenesis, tumor metastasis and migration, neuron development, and other important biological processes (17,18). Multiple studies have shown that there are many kinds of miRNA expression changes after cerebral hypoxia ischemia (19-21). It was reported that miRNA-210 induces microglial activation and regulates microglia-mediated neuroinflammation in neonatal HIE. The miRNA miR-27a has proved to implicate with many kinds of solid tumors, showing potential as a useful biomarker or drug target for clinical application (22). The ectopic expression of miR-27a was found to occur in tumor cells, and to be involved in anti-apoptosis and promoting cell proliferation (23,24). In recent years, attention has increasingly been paid to the role of miR-27a in nervous system diseases. It has been found that miR-27a-3p can prevent blood-brain barrier (BBB) damage and brain injury after intracerebral hemorrhage by targeting endothelial aquaporin-11 (25,26). In a traumatic brain injury model, miR-27a was shown to exert a neuron-protective effect by inhibiting autophagy (27). A study has also shown that miR-27a is related to microglia and the production of inflammatory mediators (28). It has also been reported that the decreased expression of miR-27b is induced by fetal distress hypoxia injury, and regulates the sensitivity of neurons to apoptosis (29).
In our previous study, the activity of hippocampal neurons was significantly decreased, and miR-27a expression was significantly decreased in the hippocampal neurons after being treated with oxygen-glucose deprivation (OGD) (30). A large number of studies have reported that apoptosis plays a very important role in hypoxic-ischemic brain damage and delayed neuronal death, especially in neonatal HIE (31). Overexpression of miR-27a could effectively reduce the decrease of cell viability and the increase of apoptosis in hippocampal neurons induced by OGD. Meanwhile, miR-27a could inhibit the expression of caspase 3 in hippocampal neurons after OGD treatment, thus playing a neuroprotective role. In this study, we firstly established a rat model of neonatal HIE, and tested the expressions of miR-27a and targeting gene FOXO1 in the brain post-HIE. Then, we investigated the protective roles of miR-27a though intracerebroventricular injection (i.c.v) of miR-27a mimic in the rat neonatal HIE model. We present the following article in accordance with the ARRIVE reporting checklist available at https://tp.amegroups.com/article/view/10.21037/tp-22-259/rc).
Methods
Rat model of neonatal HIE
According to the modified Rice-Vannucci model (32), 7-day-old (P7) Sprague-Dawley (SD) rats were used to establish the rat HIE model. These newborn rats were obtained from the laboratory animal center of Nantong University, and cultured in an operative room with room temperature controlled between 22 and 25 ℃ and a humidity of 50–70%. These P7 pups were raised independently by the maternal rats. A protocol was prepared before the study without registration. All animal experiments were approved by the Ethics Committee of Nantong University (Permission No. 20180304-007) and performed according to the Guide for the Care and Use of Laboratory Animals, 8th edition.
Pups were anesthetized with 2–3% isoflurane, and placed in the supine position with their limbs and heads fastened. A median incision in the neck was made after sterilization with 75% alcohol, and the right common carotid artery (CCA) was exposed. The CCA was double ligated and then cut between the sutures. The SD rats were placed in a self-made 1 L volume hypoxia chamber, and continuously perfused with oxygen and nitrogen mixture (oxygen concentration of 8%) 1.5 L/minute for 2 hours.
i.c.v
After the rat model of neonatal HIE was established, the rats were randomly grouped into 3 groups (sham group, miR-27a mimic group, and mimic ctrl group). Pups were fastened on a stereotaxic apparatus after being anesthetized with 2–3% isoflurane. The bregma was exposed after the skin was cut, then a miR-27a mimic or mimic ctrl (20 pmol) in a total volume of 2 µL was injected at a rate of 1 µL/minute into the ipsilateral hemisphere (2 mm posterior, 1.5 mm lateral, and 3 mm below the skull surface). After injection and skin suture, these rats were returned to their previous cages. All researchers conducting animal testing were blinded to the treatment conditions.
Real-time quantitative polymerase chain reaction
According to the product instructions, the TaqMan miRNA Isolation Kit (Thermo Fisher Scientific, Waltham, MA, USA) was applied to extract RNA for testing the expression of miR-27a from hippocampus tissues of rats in each group. After the rats were anesthetized, TRIzol reagent (Thermo Fisher, USA) was applied to isolate total RNA for testing the expression of FOXO1 mRNA from hippocampal tissues. After isolation of RNA, the RNA was quantified and qualified using a NanoDropND-1000 (Thermo Fisher, USA). Then, TaqMan microRNA Assay, TaqMan Universal PCR Master Mix, and specific primers for miR-27a and U6 were employed to test the expression of miR-27a. The primer sequences were as described in previous paper (30). Following RNA isolation using TRIzol and quantification, the total RNA was reverse transcribed into complementary DNA (cDNA), and SYBR Green (Roche, Basel, Switzerland) was adopted to test the expression of FOXO1, IL-1β, and TNF-α by real-time quantitative polymerase chain reaction (RT-qPCR) instrument LightCycler 96 (Roche, Basel, Switzerland). Glyceraldehyde 3-phosphate dehydrogenase (GAPDH) was applied as the internal reference and data were analyzed by the reported methods (30). The sequences of used primers are listed in Table S1.
Western blot
The hippocampal tissues were collected from rats, and protein lysis buffer (protease inhibitor contained) was employed to extract total protein. The protein was electrophoretically separated by sodium dodecyl sulfate polyacrylamide gel electrophoresis (SDS-PAGE) following quantification, then transferred to a polyvinylidene fluoride (PVDF) membrane. After blocking with 5% non-fat milk, the membrane was incubated with primary anti-FOXO1 antibody (1:1,000, Abcam, Cambridge, MA, USA) and anti-rat β-actin (1:2,000, Sigma-Aldrich, St. Louis, MO, USA) at 4 ℃ overnight. The membrane was reacted with HRP conjugated secondary antibodies, respectively. The relative expression of FOXO1 was represented as FOXO1/β-actin.
2,3,5-triphenytetrazolium chloride staining
The brains of pups in each group were removed and placed in pre-cooled phosphate-buffered saline (PBS) solution. These brains were stored at −20 ℃ for 30 minutes, during which time the cerebellum, olfactory bulb, and brain stem were cut. Then, 2 mm thick coronal slices were incubated in 2% 2,3,5-triphenytetrazolium chloride (TTC) solution at 37 ℃ for 15–30 minutes in the dark. Following staining, the slices were fixed in 4% paraformaldehyde (PFA), scanned, and the infarct volume was measured.
Water content in brain tissue
The water content in brain tissue was measured by dry-wet method. The cerebellum, olfactory bulb, and brain stem were cut after the brains of pups in each group had been removed. The brain right hemispheres were weighed immediately (wet weight). Then, these hemispheres were dried at 80 ℃ for 48 hours and weighed again (dry weight). The water content in brain tissue was calculated as follows: [(wet weight − dry weight)/(wet weight)] × 100%.
Single cell sorting
The methods of single cell sorting were described in previous report (33). After anesthesia, the hearts of rats were exposed, and the left ventricles were perfused with pre-cooled PBS. The olfactory bulb and brain stem were cut, followed by removal of the brain right hemispheres. The hemispheres were transferred into pre-cooled Dulbecco’s modified Eagle medium (DMEM) solution and cut into pieces, then digested with DNAse (1 mg/mL) and collagenase (1 mg/mL) for 45 minutes at 37 ℃. Through a 70 µm cell strainer, the single cell suspension was centrifuged and re-suspended in 70% Percoll solution. Following overlay with 37% Percoll, the solution was centrifuged at 500 g for 20 minutes, and taken the interphase between 70% and 37% phase into a fresh tube. After washing, all isolated cells were resuspended in 1 mL PBS containing 1% fetal bovine serum (FBS), and stained with Trypan blue to count.
Briefly, single cells were incubated with Fc receptor blocking solution for 15 minutes, and immunostained with combinations of different surface antibodies. The antibodies used are shown in Table S2. The gating strategy was as follows: peripheral lymphocytes (CD45hi+), T cells (CD45+ CD3+CD19−), B cells (CD45+CD3−CD19+), natural killer (NK) cells (CD45+CD3−NK1.1+), macrophage (CD45hi+CD11b+F4/80+), and neutrophil (CD45hi+CD11b+Ly6G+). Flow cytometry (FCM) data were acquired on FACS Canto (BD Biosciences, San Jose, CA, USA) and analyzed using FlowJo software (BD, USA).
Statistical analysis
Data were presented as means ± standard deviation (SD) and analyzed using GraphPad Prism 8 software (GraphPad Software, La Jolla, CA, USA). The unpaired Student’s t-test and one-way analysis of variance (ANOVA) were used to compare differences in groups. A P value <0.05 was considered statistically significant.
Results
The expressions of miR-27a and FOXO1 in the brain after HIE
We used RT-qPCR to test the expressions of miR-27a and FOXO1 mRNA in hippocampal tissues after HIE in neonatal rats. In the previous report, it was identified that FOXO1 was the target gene of miR-27a. The results showed that the expressions of miR-27a were decreased gradually after HIE; however, the expressions of FOXO1 mRNA were increased, compared to sham group. The results are shown in Figure 1. However, after i.c.v with miR-27a mimic for 48 hours, the expression of miR-27a was significantly upregulated (P<0.01) compared to the mimic ctrl group. As we anticipated, the expression of FOXO1 mRNA was significantly downregulated (P<0.01). Western blot was used to further test the expression of FOXO1 after injection with miR-27a mimic. Compared with the mimic ctrl group, the expression of FOXO1 was robustly decreased (Figure 2). These results showed that miR-27a mimic could promote the expression of miR-27a but inhibit the expression of FOXO1 in hippocampus.
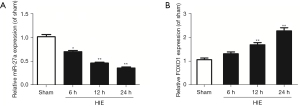
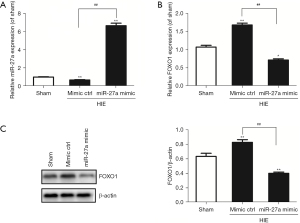
MiR-27a reduced infarcted volume and brain edema after HIE
The TTC staining was applied to test the effect of miR-27a on the infarcted volume after HIE. The staining results showed that the infarct volume was markedly increased (P<0.01) compared to the sham group. Interestingly, the infarct volume in the miR-27a mimic group was obviously reduced (P<0.05) compared with the mimic ctrl group (Figure 3A).
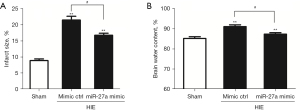
Brain water content measurement was adopted to test the effect of miR-27a on brain edema after HIE. Compared to the sham group, the brain water contents in the HIE groups were significantly raised (P<0.01). However, compared to the mimic ctrl group, the brain water content in the miR-27a group was obviously reduced (P<0.05) (Figure 3B). The above results indicated that miR-27a could reduce the infarct volume and edema after HIE.
MiR-27a reduced inflammatory factors after HIE
We applied RT-qPCR to test the expressions of inflammatory factors IL-1β and TNF-α post-HIE. The results of RT-qPCR showed that the expressions of inflammatory factors (IL-1β and TNF-α) post-HIE were upregulated (P<0.01) compared to the sham group. However, the expressions of inflammatory factors after injection of miR-27a were apparently downregulated (P<0.05) compared to the mimic ctrl group (Figure 4). These results potentially indicated that miR-27a played protective roles by alleviating productions of pro-inflammatory cytokines post-HIE.
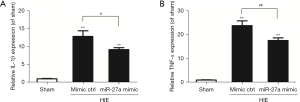
miR-27a decreased infiltrated immune cells after HIE
Following HIE, alongside the upregulation of the expressions of inflammatory factors, the infiltrated peripheral leukocytes were also increased for neuroinflammation. So, we tested the effects of miR-27a on the infiltrated peripheral leukocytes by using FCM analysis. The infiltrated peripheral T, B, and NK cell numbers were not different in 3 groups (Figure 5). This may have been due to too few T, B, and NK cells in the brain post-HIE. We also tested the effects of miR-27a on peripheral monocytes/macrophages and neutrophils (CD45hi+CD11b+). The results of FCM analysis showed that the peripheral monocytes (CD45hi+CD11b+) cell numbers were significantly increased (P<0.01) compared to in the sham group. Besides the cell numbers of macrophages (CD45hi+CD11b+F4/80+) and neutrophils (CD45hi+CD11b+LY6G+) were all increased post-HIE. Interestingly, after injection of the miR-27a mimic, the numbers of peripheral monocytes, macrophages, and neutrophils were all apparently decreased (P<0.05), compared to in the mimic ctrl group (Figure 6). These results illustrated that miR-27a could inhibit the infiltration of peripheral monocytes post-HIE.
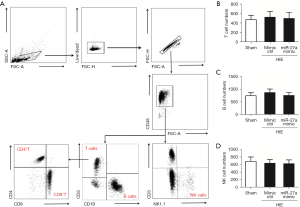
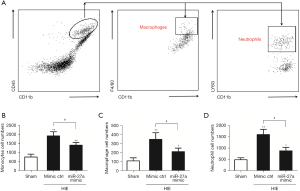
Discussion
The treatment strategy for HIE is currently being explored. To date, mild hypothermia is the only effective treatment method (34). Approximately 40–50% of HIE children still die or experience neurological sequelae after mild hypothermia treatment, which brings a heavy burden to their family and society (34). Therefore, researchers have sought to explore the pathogenesis of HIE and develop new treatment options for neonatal HIE for many years.
The FOXO1 gene is an important transcription factor that regulates oxidative stress, cell proliferation, and apoptosis, and is widely expressed in liver, fat, brain, and skeletal muscle, among other tissues (30,35). The expression of FOXO1 was found in the first layer, pyramidal cell layer, and radiation layer of the CA1 area in the damaged hippocampus in the model of cerebral ischemia (29,30). Moreover, FOXO1 was activated first, followed by delayed neuronal death, in the injured hippocampus caused by focal cerebral ischemia, suggesting that the signaling pathway of FOXO1 was involved in the neuronal death (30). Some studies have also found that FOXO transcription factor plays an important role in regulating lymphocyte specific function and maintaining T cell silence (36,37). Both FOXO1 and FOXO3 are primary targets of the PI3K/Akt pathway in T cells, which play a key role in maintaining T cell silence, controlling growth factors, and inflammatory response (37,38).
In our previous study, the existence of a direct interaction between miR-27a and FOXO1 and the interaction sites has been confirmed. By using miR-27a mimics, overexpression of miR-27a in hippocampal neurons significantly inhibited the expression of FOXO1 to reduce OGD-induced apoptosis in hippocampal neurons (30). Meanwhile, overexpression of FOXO1 could reverse the cellular neuronal protective effect induced by overexpression of miR-27a in hippocampal neurons after OGD treatment, suggesting that miR-27a plays a protective role in hippocampal neurons by targeting FOXO1 (30). In this paper, we firstly established a rat model of neonatal HIE, and tested the expressions of miR-27a and targeting gene FOXO1 in the brain post-HIE. The expressions of miR-27a were decreased gradually after HIE; however, the expressions of FOXO1 mRNA were increased. In order to investigate the protective roles of miR-27a in HIE, miR-27a was overexpressed by i.c.v with an miR-27a mimic. As anticipated, the expression of miR-27a in the brain was significantly upregulated; however, the expression of FOXO1 was robustly downregulated after injection of the miR-27a mimic.
It is well known that microglia are innate immune cells in the brain, which can cause inflammatory reactions under neuropathological conditions, such as perinatal HIE, infection, and traumatic brain injury, as well as in autoimmune and neurodegenerative diseases (39). Mounting evidence shows that inflammatory cells play an important role in the remodeling and repair of acute brain injury (40). The neuroinflammatory response after acute hypoxic-ischemic brain injury was characterized by the activation of microglia, and the infiltration of peripheral macrophages, monocytes and neutrophils into the brain, and the release of cytokines and chemokines by inflammatory cells (41). Therefore, we speculate that FOXO1 can regulate inflammation by affecting lymphocyte infiltration in HIE to play a neuron-protective role. In this paper, we investigated the protective effects of miR-27a by TTC staining, brain water content measurement, and FCM analysis. It was revealed that miR-27a could significantly reduce the infarcted volume and brain edema. Importantly, miR-27a could effectively inhibit infiltrated peripheral monocytes (CD45hi+CD11b+), macrophages (CD45hi+CD11b+F4/80+), and neutrophils (CD45hi+CD11b+LY6G+) into the brain after HIE. Besides, miR-27a also could alleviate the expressions of IL-1β mRNA and TNF-α mRNA. Obviously, miR-27a played a protective function by inhibiting HIE-induced neuroinflammatory response.
In summary, miR-27a plays protective roles by reducing infarct volume and brain edema, and inhibiting inflammatory factors and infiltrated peripheral immune cells by targeting FOXO1 in neonatal HIE rats. In the future, miR-27a could be applied as a treatment strategy for HIE.
Acknowledgments
We are very thankful for the technological support of the Key Laboratory for Neuroregeneration of Nantong University.
Funding: The study was supported by the Natural Science Research Project of Nantong Science and Technology Bureau (No. JCZ19031 and MSZ20078).
Footnote
Reporting Checklist: The authors have completed the ARRIVE reporting checklist. Available at https://tp.amegroups.com/article/view/10.21037/tp-22-259/rc
Data Sharing Statement: Available at https://tp.amegroups.com/article/view/10.21037/tp-22-259/dss
Conflicts of Interest: All authors have completed the ICMJE uniform disclosure form (available at https://tp.amegroups.com/article/view/10.21037/tp-22-259/coif). The authors have no conflicts of interest to declare.
Ethical Statement: The authors are accountable for all aspects of the work in ensuring that questions related to the accuracy or integrity of any part of the work are appropriately investigated and resolved. All animal experiments were approved by the Ethics Committee of Nantong University (Permission No. 20180304-007) and performed according to the
Open Access Statement: This is an Open Access article distributed in accordance with the Creative Commons Attribution-NonCommercial-NoDerivs 4.0 International License (CC BY-NC-ND 4.0), which permits the non-commercial replication and distribution of the article with the strict proviso that no changes or edits are made and the original work is properly cited (including links to both the formal publication through the relevant DOI and the license). See: https://creativecommons.org/licenses/by-nc-nd/4.0/.
References
- Russ JB, Simmons R, Glass HC. Neonatal Encephalopathy: Beyond Hypoxic-Ischemic Encephalopathy. Neoreviews 2021;22:e148-62. [Crossref] [PubMed]
- Domoki F. Hydrogen-induced Neuroprotection in Neonatal Hypoxic-ischemic Encephalopathy. Curr Pharm Des 2021;27:687-94. [Crossref] [PubMed]
- Greco P, Nencini G, Piva I, et al. Pathophysiology of hypoxic-ischemic encephalopathy: a review of the past and a view on the future. Acta Neurol Belg 2020;120:277-88. [Crossref] [PubMed]
- Gao L, Yang L, Cui H. GSK-3beta inhibitor TWS119 alleviates hypoxic-ischemic brain damage via a crosstalk with Wnt and Notch signaling pathways in neonatal rats. Brain Res 2021;1768:147588. [Crossref] [PubMed]
- Shao R, Sun D, Hu Y, et al. White matter injury in the neonatal hypoxic-ischemic brain and potential therapies targeting microglia. J Neurosci Res 2021;99:991-1008. [Crossref] [PubMed]
- Zhu X, Yan J, Bregere C, et al. RBM3 promotes neurogenesis in a niche-dependent manner via IMP2-IGF2 signaling pathway after hypoxic-ischemic brain injury. Nat Commun 2019;10:3983. [Crossref] [PubMed]
- Barks JDE, Liu Y, Dopp IA, et al. Azithromycin reduces inflammation-amplified hypoxic-ischemic brain injury in neonatal rats. Pediatr Res 2021; Epub ahead of print. [Crossref] [PubMed]
- Shu J, Jiang L, Wang M, et al. Human bone marrow mesenchymal stem cells-derived exosomes protect against nerve injury via regulating immune microenvironment in neonatal hypoxic-ischemic brain damage model. Immunobiology 2022;227:152178. [Crossref] [PubMed]
- Ovcjak A, Xiao A, Kim JS, et al. Ryanodine receptor inhibitor dantrolene reduces hypoxic-ischemic brain injury in neonatal mice. Exp Neurol 2022;351:113985. [Crossref] [PubMed]
- Yang L, Zhao H, Cui H. Treatment and new progress of neonatal hypoxic-ischemic brain damage. Histol Histopathol 2020;35:929-36. [PubMed]
- Tetorou K, Sisa C, Iqbal A, et al. Current Therapies for Neonatal Hypoxic-Ischaemic and Infection-Sensitised Hypoxic-Ischaemic Brain Damage. Front Synaptic Neurosci 2021;13:709301. [Crossref] [PubMed]
- McDouall A, Wassink G, Bennet L, et al. Challenges in developing therapeutic strategies for mild neonatal encephalopathy. Neural Regen Res 2022;17:277-82. [Crossref] [PubMed]
- Chalak LF, Adams-Huet B, Sant'Anna G. A Total Sarnat Score in Mild Hypoxic-ischemic Encephalopathy Can Detect Infants at Higher Risk of Disability. J Pediatr 2019;214:217-221.e1. [Crossref] [PubMed]
- O'Mara K, McPherson C. Neuroprotective Agents for Neonates with Hypoxic-Ischemic Encephalopathy. Neonatal Netw 2021;40:406-13. [Crossref] [PubMed]
- Wang W, Jia L. Regulatory Mechanism of MicroRNA-30b on Neonatal Hypoxic-Ischemic Encephalopathy (HIE). J Stroke Cerebrovasc Dis 2021;30:105553. [Crossref] [PubMed]
- Islam MR, Kaurani L, Berulava T, et al. A microRNA signature that correlates with cognition and is a target against cognitive decline. EMBO Mol Med 2021;13:e13659. [Crossref] [PubMed]
- Meng Q, Yang P, Lu Y. MicroRNA-410 serves as a candidate biomarker in hypoxic-ischemic encephalopathy newborns and provides neuroprotection in oxygen-glucose deprivation-injured PC12 and SH-SY5Y cells. Brain Behav 2021;11:e2293. [Crossref] [PubMed]
- Morris G, O'Brien D, Henshall DC. Opportunities and challenges for microRNA-targeting therapeutics for epilepsy. Trends Pharmacol Sci 2021;42:605-16. [Crossref] [PubMed]
- Paul S, Bravo Vázquez LA, Pérez Uribe S, et al. Current Status of microRNA-Based Therapeutic Approaches in Neurodegenerative Disorders. Cells 2020;9:1698. [Crossref] [PubMed]
- Eyileten C, Sharif L, Wicik Z, et al. The Relation of the Brain-Derived Neurotrophic Factor with MicroRNAs in Neurodegenerative Diseases and Ischemic Stroke. Mol Neurobiol 2021;58:329-47. [Crossref] [PubMed]
- Chen YM, He XZ, Wang SM, et al. delta-Opioid Receptors, microRNAs, and Neuroinflammation in Cerebral Ischemia/Hypoxia. Front Immunol 2020;11:421. [Crossref] [PubMed]
- Zhang J, Cao Z, Yang G, et al. MicroRNA-27a (miR-27a) in Solid Tumors: A Review Based on Mechanisms and Clinical Observations. Front Oncol 2019;9:893. [Crossref] [PubMed]
- Lu X, Kang N, Ling X, et al. MiR-27a-3p Promotes Non-Small Cell Lung Cancer Through SLC7A11-Mediated-Ferroptosis. Front Oncol 2021;11:759346. [Crossref] [PubMed]
- Chen H, Zhang Y, Cao X, et al. MiR-27a Facilitates Breast Cancer Progression via GSK-3beta. Technol Cancer Res Treat 2020;19:1533033820965576. [Crossref] [PubMed]
- Zhou Q, Feng X, Ye F, et al. miR-27a promotion resulting from silencing of HDAC3 facilitates the recovery of spinal cord injury by inhibiting PAK6 expression in rats. Life Sci 2020;260:118098. [Crossref] [PubMed]
- Florian IA, Buruiana A, Timis TL, et al. An Insight into the microRNAs Associated with Arteriovenous and Cavernous Malformations of the Brain. Cells 2021;10:1373. [Crossref] [PubMed]
- Li J, Peng L, Bai W, et al. Biliverdin Protects Against Cerebral Ischemia/Reperfusion Injury by Regulating the miR-27a-3p/Rgs1 Axis. Neuropsychiatr Dis Treat 2021;17:1165-81. [Crossref] [PubMed]
- Zhao Q, Lu F, Su Q, et al. Knockdown of long noncoding RNA XIST mitigates the apoptosis and inflammatory injury of microglia cells after spinal cord injury through miR-27a/Smurf1 axis. Neurosci Lett 2020;715:134649. [Crossref] [PubMed]
- Li W, Zhu Q, Xu X, et al. MiR-27a-3p suppresses cerebral ischemia-reperfusion injury by targeting FOXO1. Aging (Albany NY) 2021;13:11727-37. [Crossref] [PubMed]
- Cai Q, Wang T, Yang WJ, et al. Protective mechanisms of microRNA-27a against oxygen-glucose deprivation-induced injuries in hippocampal neurons. Neural Regen Res 2016;11:1285-92. [Crossref] [PubMed]
- Rodriguez J, Li T, Xu Y, et al. Role of apoptosis-inducing factor in perinatal hypoxic-ischemic brain injury. Neural Regen Res 2021;16:205-13. [Crossref] [PubMed]
- Vannucci RC, Vannucci SJ. Perinatal hypoxic-ischemic brain damage: evolution of an animal model. Dev Neurosci 2005;27:81-6. [Crossref] [PubMed]
- Jiang M, Li R, Lyu J, et al. MCC950, a selective NLPR3 inflammasome inhibitor, improves neurologic function and survival after cardiac arrest and resuscitation. J Neuroinflammation 2020;17:256. [Crossref] [PubMed]
- Finder M, Boylan GB, Twomey D, et al. Two-Year Neurodevelopmental Outcomes After Mild Hypoxic Ischemic Encephalopathy in the Era of Therapeutic Hypothermia. JAMA Pediatr 2020;174:48-55. [Crossref] [PubMed]
- Remadevi V, Muraleedharan P, Sreeja S. FOXO1: a pivotal pioneer factor in oral squamous cell carcinoma. Am J Cancer Res 2021;11:4700-10. [PubMed]
- Xu K, Yin N, Peng M, et al. Glycolysis fuels phosphoinositide 3-kinase signaling to bolster T cell immunity. Science 2021;371:405-10. [Crossref] [PubMed]
- Dudek M, Pfister D, Donakonda S, et al. Auto-aggressive CXCR6+ CD8 T cells cause liver immune pathology in NASH. Nature 2021;592:444-9. [Crossref] [PubMed]
- Seda V, Vojackova E, Ondrisova L, et al. FoxO1-GAB1 axis regulates homing capacity and tonic AKT activity in chronic lymphocytic leukemia. Blood 2021;138:758-72. [Crossref] [PubMed]
- Absinta M, Maric D, Gharagozloo M, et al. A lymphocyte-microglia-astrocyte axis in chronic active multiple sclerosis. Nature 2021;597:709-14. [Crossref] [PubMed]
- Lv Y, Sun B, Lu XX, et al. The role of microglia mediated pyroptosis in neonatal hypoxic-ischemic brain damage. Biochem Biophys Res Commun 2020;521:933-8. [Crossref] [PubMed]
- Chen HR, Chen CW, Kuo YM, et al. Monocytes promote acute neuroinflammation and become pathological microglia in neonatal hypoxic-ischemic brain injury. Theranostics 2022;12:512-29. [Crossref] [PubMed]